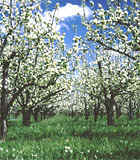 
|
Contributed by Patricia McManus University of Madison-Wisconsin
Virginia Stockwell Oregon State University
McManus, P. and Stockwell, V. 2000. Antibiotics for Plant Diseases Control: Silver Bullets or Rusty Sabers. APSnet Features. Online. doi: 10.1094/APSnetFeature-2000-0600
|
At sunrise, the perfect spring dawn reveals pear blossoms, fully opened, filling the calm morning air with a fresh but pungent scent. Occasional petals rain confetti to the orchard floor. As morning chill gives way to warmth, the new day hums with bees actively foraging and pollinating the blossoms. Conditions are ideal for a good crop. Unseen lurks a bacterium, which thrives in these same conditions and in a matter of days, will ruin the potential for a pear harvest. A distant rumble grows louder as a tractor approaches; the driver is imposing and alien in her white spray suit. A sprayer blasts an antibiotic-laden mist into the blooming canopy. Moments later the tractor is gone and the tranquility of the spring morning resettles in the orchard. Was this interruption the climax of a horror film or a farmer’s only hope for a crop?
In the 1950s, soon after the introduction of antibiotics in human medicine, the potential of these "miracle drugs" to work wonders in controlling plant diseases was explored. Nearly 40 antibiotics were screened for plant disease control (Goodman 1959). To be a viable candidate for disease control, the antibiotic needed to: (i) be active on or inside of the plant; (ii) tolerate oxidation, UV irradiation, rainfall, and high temperatures; (iii) be safe to plants; and (iv) select for resistant pathogens only at a low or non-detectable rate. Of the screened compounds, fewer than 10 were used commercially and only streptomycin saw significant use worldwide. For the control of certain bacterial diseases of plants, streptomycin was indeed a silver bullet.
Unfortunately, just as the emergence of bacterial strains resistant to antibiotics has limited their performance in clinical settings, streptomycin resistance has destabilized plant disease control. The antibiotic resistance crisis in medicine has been widely publicized and is recognized as a major threat to controlling human bacterial diseases and infections worldwide (Levy 1992; Alliance for the Prudent Use of Antibiotics, http://www.apua.org). Efforts to conserve the efficacy of antibiotics in medicine have drawn scrutiny to all antibiotic use within and outside the medical field, including use on crop plants. Concern from the general public and within the scientific community has resulted in a number of misconceptions regarding antibiotic use on plants. This article describes the current scenario regarding antibiotic use on plants in the U.S., the emergence of antibiotic resistant plant pathogens, and the recent political upheaval regarding antibiotic use and resistance.
Battling bacterial pathogens: How much damage can the little bugs do?
As is the case in human and veterinary medicine, bacteria and other prokaryotic microbes (e.g., phytoplasmas) are the target of antibiotic use on plants. As a group, bacteria and phytoplasmas have been documented to cause fewer diseases of plants than do fungi and viruses (Table1).
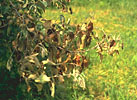 |
|
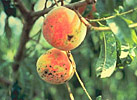 |
Click on any image above for an enlargement and more information. |
Although bacterial diseases are relatively few, they can cause dire economic losses. For example, bacterial soft rots of fruits and vegetables cause an estimated 50 to 100 million dollars in losses per year worldwide (Perombelon and Kelman 1980). Efforts to eradicate Citrus canker caused by Xanthomonas campestris pv. citri from the Gulf States resulted in the destruction of over 20 million trees in nurseries or groves, and the problem has not been resolved. It should be noted that antibiotics are not used to control most bacterial diseases of plants, including Citrus canker.
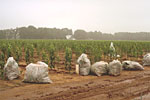 |
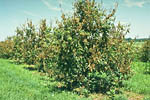 |
Click on either image above for enlargement and more information. |
Bacterial diseases of plants are very difficult to manage. In addition to visible disease symptoms, bacteria can become systemic in a plant’s vascular tissue making it impractical to eradicate the pathogen by pruning out symptomatic tissues or by applying a pesticide to the plant surface. Moreover, bacteria undergo exponential growth, meaning that their populations can double several times daily, depending on the bacterial species and environmental conditions. Thus, bacterial diseases are explosive; by the time symptoms are recognized, the pathogen often is entrenched and well on its way to destroying the crop.
Managing bacterial diseases depends mostly on host resistance (which may not be available in desirable crop varieties), sanitation (e.g., preventing the introduction of pathogens and removing diseased plants), and cultural practices (e.g., avoiding overhead irrigation and limiting nitrogen fertilization). In some cases, chemical bactericides (e.g., copper compounds and antibiotics) and biological control agents are effectively integrated into the disease management program. This article focuses on the role of antibiotics in plant disease control, with emphasis on fire blight of apple and pear, the target of greater than 90% of the use of antibiotics on crop plants.
Just the facts: Who, what, where, why, how, and to what extent?
What are antibiotics and which antibiotics are used on plants? The classic definition of an antibiotic is a compound produced by a microorganism that inhibits the growth of another microorganism. Over the years, this definition has been expanded to include synthetic and semi-synthetic products. Antibiotics are grouped based on chemical structure or the mechanism of inhibition of microorganisms. Table 2 illustrates the major classes of antibiotics and their uses in crop protection, animal agriculture, and human medicine. Of the myriad antibiotics used in agriculture, only two compounds are used on crop plants in the U.S.: streptomycin and oxytetracycline.
Streptomycin. In the U.S., streptomycin use is permitted on 12 plant species, but the primary uses are on apple, pear, and related ornamental trees for the control of fire blight caused by Erwinia amylovora (Table 3). Minor uses include floriculture, seed treatment, and on seedlings of celery, pepper, potato, tomato, and tobacco in the greenhouse and/or field. At high concentrations, streptomycin can be phytotoxic to plants; thus it is applied to the surface of plants and not injected.
Oxytetracycline. This antibiotic is used primarily on peach, nectarine, and pear (Table 3). Oxytetracycline also is used on an emergency basis on apple in specific regions where streptomycin-resistant strains of E. amylovora have been documented. Tetracycline derivatives are the only antibiotics that also can be used internally in plants. Tetracyclines are injected into the trunks of palm and elm trees to treat lethal yellows diseases caused by phytoplasmas (Agrios 1997). The injection of tetracycline into a tree is a labor-intensive and expensive treatment, which often must be repeated for abatement or delay of symptoms. Antibiotic injections are practical only for isolated high-value ornamental trees and not vast plantings of agricultural or forest trees.
What quantities of antibiotics are used on plants? In 1997, approximately 30,800 pounds of streptomycin and 26,700 pounds of oxytetracycline were applied to fruit trees in the major tree-fruit producing states (Tables 3, 4, 5; NASS 1997). This accounts for about 0.1% of total annual antibiotic use in the U.S. Referring to pounds of antibiotics produced or used is a bit overwhelming and incomprehensible. For example, the Centers for Disease Control and Prevention (CDC) estimates that 50 million pounds of antibiotics are produced annually in the U.S. and translates this into 230 million doses of antibiotics consumed by humans. The following figures may help depict antibiotic use on plants. Nationwide in 1997, antibiotics were applied once or twice to 25% of apple acreage, four or five times to about 40% of pear acreage, and four times to 8% of peach and nectarine acreage (Table 3; NASS 1997). Not included in these figures is the sale of streptomycin in small quantities to home gardeners. This probably represents a tiny fraction of total sales but would be a significant source of exposure to antibiotics if the purchaser were careless in handling the product.
In commercial orchards, antibiotic powders are mixed in a large volume of water to a concentration of 50-200 parts per million (ppm) and blown into tree canopies with a high- pressure (air-blast) sprayer pulled by a tractor |
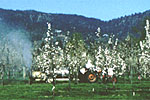 |
Regulation of antibiotic use on plants
Facts on Antibiotic Use on Plants in the U.S.
Two antibiotics (streptomycin and oxytetracycline) are used on plants.
Use on plants accounts for about 0.1% of total antibiotic use.
Antibiotic use on plants is regulated by the Environmental Protection Agency.
Antibiotics are applied to about 25% of apple acreage; 35-40% of pear acreage; and 8% of peach and nectarine acreage.
Controlling fire blight of apple, pear, and related ornamental plants accounts for the majority of antibiotic use on plants.
|
Like all pesticides, antibiotic use on crops and ornamental plants is regulated in the U.S. by the Environmental Protection Agency (EPA). The registration process involves evaluation of the toxicity and carcinogenicity of a product or its derivatives and also impacts that a chemical may have on non-target species including plants, pollinating insects, aquatic organisms, and other wildlife. Regulatory decisions are not based on the efficacy of disease control. In comparison to most pesticides, streptomycin and oxytetracycline are relatively non-toxic and have been assigned the lowest toxicity rating of the EPA, category IV (EPA R.E.D. fact sheets). |
The use of pesticides on certified organic crops is regulated by state or regional certification programs, and national standards are under development. In certified organic farming systems, the use of antibiotics is strictly limited to pear and apple for the control of the bacterial disease fire blight (http://www.ams.usda.gov/nop for national program, http://agr.wa.gov/FoodAnimal/Organic/ for Washington state standards, and http://www.ccof.org/ for California standards). According to the Organic Materials Review Institute, streptomycin and oxytetracycline are included in the national list of permitted materials as regulated materials. "Regulated materials" are those that can be used if no alternatives are feasible and efforts to reduce use of such materials are included in the farm plan. Antibiotics are not permitted on any other crops in certified organic fields.
Product labels and supplemental literature state what type of clothing, gloves, and respirators must be worn by mixers, applicators, and persons entering an area shortly after antibiotics have been applied. Re-entry into the sprayed area without protective gear is forbidden for 12 hours after using streptomycin or oxytetracycline. It is a violation of federal law to use any pesticide, including antibiotics, in a manner inconsistent with its labeling.
Product labels also specify a preharvest interval (PHI), the period of time between the last permitted application and harvest. PHIs are established to ensure that pesticide residues do not exceed a predetermined limit or "tolerance." The PHIs for oxytetracyline and streptomycin range from 21 to 60 days, depending on the crop and antibiotic. Residue tolerances of 0.25 ppm and 0.35 ppm of streptomycin and oxytetracycline, respectively, on produce have been established by the EPA based on toxicology tests.
Fire blight: The primary target for antibiotic use on plants
In 1998, apple and pear growers in Washington and northern Oregon suffered an estimated 68 million dollars in losses due to outbreaks of fire blight caused by E. amylovora. Since 1997, approximately 500,000 pear trees have been destroyed in the Po Valley of Italy, which is the major pear production area of the world, in an effort to eradicate fire blight (Calzolari et al. 1999). Another 580,000 pear, apple, and quince trees were destroyed in Romania between 1993 to 1997 and 340,000 pear and apple trees were destroyed in Croatia since 1995 in efforts to halt the spread of fire blight in those countries (Cvjetkovic et al. 1999, Severin et al. 1999).
|
|
Click on any image above for enlargement and more information. |
An apple or pear orchard may see little fire blight for several years and then succumb to an epidemic when environmental conditions favorable to the disease coincide with bloom (van der Zwet and Beer 1991). Fire blight is broken down into several subcategories such as blossom blight, shoot blight and rootstock blight, each named for the tissue that is infected and killed. Additionally, "trauma blight" can occur on shoots and fruits due to wounds from hailstorms or wind. Blossom blight is the phase best controlled by antibiotics (van der Zwet and Beer 1991). In the U.S., blossom blight is most common in the western states on pear, whereas shoot and rootstock blight have caused devastating losses in apple orchards throughout the U.S. and Europe.
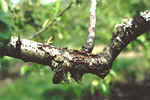 |
Click on image for more information. |
In order to understand fire blight control strategies, a basic understanding of the disease is needed. The pathogen, E. amylovora, overwinters in cankers or infected tissues from the previous season. In the spring, as temperatures increase, the pathogen multiplies in the cankers and spreads to open blossoms. When temperatures exceed 15° C (60° F), the pathogen multiplies rapidly on the stigmas of blossoms. After the population size of the pathogen exceeds about one million cells per blossom, infection occurs. After infection, the pathogen grows internally in the plant causing cell death, which is seen as wilting and blackening of the infected tissues. Once these symptoms are visible, there is no effective chemical treatment for fire blight. To minimize further disease spread, the grower must prune branches at least 12 inches below the visible symptoms. Depending on where infection occurs on a tree, the result can be the loss of major fruit-bearing limbs or the entire tree.
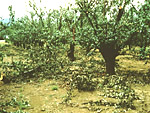 |
Click on image for more information. |
Fire blight management is multifaceted, with antibiotics being just one component (Johnson and Stockwell 1998, van der Zwet and Beer 1991). Sanitation, the removal of diseased tissues, is the most important practice for fire blight control. Pre-bloom copper sprays also can decrease populations of the pathogens at canker margins, but copper generally is not used after bloom due to phytotoxicity that results in blemished fruit. While all commercial pear varieties are susceptible to the disease, genetic resistance exists in apple cultivars such as Red Delicious and Winesap. However, consumer demand determines which apple varieties are produced and many newer popular varieties, such as Gala, Fuji, Braeburn, Honeycrisp, and Pink Lady, are highly susceptible to fire blight (van der Zwet and Beer 1991). Furthermore, many semi-dwarfing apple rootstocks, which have desirable horticultural characteristics, are very sensitive to fire blight. Infections of blossoms or shoots can spread internally to the rootstock and kill the tree rapidly (van der Zwet and Beer 1991).
Antibiotics are effective during the growth phase of the pathogen on the surface of flowers before infection. Streptomycin has been the antibiotic of choice as it kills the pathogen (bactericidal). Oxytetracycline, which stops the growth of pathogen but does not kill existing populations (bacteristatic), is less effective than streptomycin (McManus and Jones 1994). The length of time that an antibiotic is active on the surface of the flower is not well understood, but generally streptomycin is considered active for 3 days and oxytetracycline for 1 day after application. Timing is critical to the use of either antibiotic for fire blight control: unnecessary sprays are expensive, but an omitted spray during an infection period can set the stage for an epidemic.
EPA-approved product labels recommend spraying antibiotics for fire blight control on a calendar basis, beginning during bloom and repeating every 3 to 5 days until a specified time before harvest. With that schedule, up to 15 streptomycin sprays and 10 oxytetracycline sprays could be applied. If every acre of pear were sprayed at the maximum dose, then 264,810 pounds of streptomycin and 88,270 pounds of oxytetracycline would be applied annually. Spraying every acre of apple at the maximum dose would result in application of 802,455 pounds of streptomycin and 400,221 pounds of oxytetracycline every year. Comparing these figures to those shown in Table 3 makes it clear that growers are actually using less than 5% of the amount of antibiotic permitted.
Most growers are prudent in their use of antibiotics because (i) antibiotics are very expensive (about $25 to $30 per acre for the product and an additional $20 application expense); (ii) growers wish to minimize selection pressure for antibiotic resistant strains of the pathogen (see below); and (iii) research has demonstrated that there is little or no benefit to spraying later than the bloom period. Growers rely on recommendations of university-based Extension personnel and disease-risk models to optimize the timing of antibiotic sprays and to minimize the number of applications. Disease-risk models for fire blight evaluate weather factors to predict if conditions are favorable for the disease and if antibiotics should be sprayed (e.g. COUGARBLIGHT http://www.ncw.wsu.edu/treefruit/fireblight/2000f.htm; MARYBLYT http://www.ca.uky.edu/agcollege/plantpathology/ext_files/ppfshtml/ppfs-fr-t-7.pdf; for a comparison of several models see: http://www.ipm.ucdavis.edu/DISEASE/DATABASE/fireblight.html). While none of the models are perfect in predicting when and where fire blight will occur, they are useful management tools that have spared growers and the environment many antibiotic sprays.
Data from individual states illustrates shifts in antibiotic use on apple and pear in the 1990s (Tables 4 and 5). Growers in most major apple-producing states sprayed streptomycin an average of two times per year, but the percentage of acreage that was treated varied greatly among states (Table 4). Although alternating sprays of streptomycin and oxytetracycline might alleviate the selection for resistance to either or both antibiotics, the practice of EPA has been to grant special registration of oxytetracycline on apple only after streptomycin resistance in E. amylovora has been confirmed. Thus oxytetracycline is used on apple only on a limited basis in areas where streptomycin-resistant strains of E. amylovora have been documented. An increase of apple acreage treated with antibiotics during the 1990s may reflect the increased planting of popular apple varieties that are highly susceptible to fire blight.
A greater percentage of pear acreage was sprayed with antibiotics during the 1990s compared with apple acreage (Table 5). This is probably because of the greater inherent susceptibility of pear to fire blight compared to apple and the tendency of pear to remain in bloom for prolonged periods, especially in California.
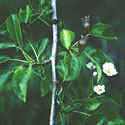 |
Click on image for more information. |
Secondary blossoms or flowers that open after fruit are developing, are common in California orchards and are particularly vulnerable to fire blight, thus requiring additional antibiotic sprays. After Extension educators publicized the prevalence of streptomycin-resistant strains of E. amylovora in Washington (Loper et al. 1991), streptomycin was rarely used on pear in that state. Many pear growers have adopted oxytetracycline, or combinations of oxytetracycline and streptomycin for fire blight control.
Biological control arrived on the fire blight control scene relatively recently. A naturally occurring, non-pathogenic bacterium, Pseudomonas fluorescens strain A506 (BlightBan A506, Plant Health Technologies, Fresno, CA) was used on 30% of the pear acreage in California in 1997 and 18% of the apple acreage in Washington in 1997 (NASS 1997). The biocontrol bacterium competes with the pathogen for nutrients and space on the stigmas of flowers. The competition from the biocontrol bacterium keeps the pathogen from reaching large population sizes, decreasing the number of infected blossoms by about half. Biocontrol can be integrated into antibiotic spray programs (Stockwell et al. 1996a). The combination of the biocontrol and streptomycin provides greater control than either implemented alone (Lindow et al. 1996). Unlike antibiotics, which lose effectiveness over a few days, biocontrol bacteria multiply and persist on blossoms. It is too early to determine the impact of biological control on management practices of fire blight. However, at least in theory, using biological control bacteria will reduce selection for antibiotic-resistant strains of E. amylovora by reducing pathogen populations and the number of antibiotic sprays needed.
Antibiotic resistance: The silver bullet is tarnished
Although the amount of antibiotics used on plants is meager compared to medical and veterinary uses, streptomycin-resistant plant pathogens have emerged (Table 6), which further complicates control of bacterial diseases of plants. Surveillance for antibiotic-resistant plant pathogens has been sporadic and usually in response to a disease control failure in the field. Many, if not most, surveys for streptomycin-resistant E. amylovora have come up negative; Table 6 reports only positive results. Surveys have not detected oxytetracycline resistance in E. amylovora (Loper et al 1991; Palmer and Jones 1999) but have identified tetracycline resistance genes in nonpathogenic bacteria in apple orchards (Palmer and Jones 1999).
In E. amylovora, two genetically distinct types of streptomycin resistance have been described (Table 7; Chiou and Jones 1995a,b): (i) a point mutation in the chromosomal gene rpsL which prevents streptomycin from binding to its ribosomal target; and (ii) inactivation of streptomycin by a phosphotransferase, encoded by strA and strB. The chromosomal mechanism occurs naturally in populations of E. amylovora at a rate of about 1 in 10 billion cells. The genes strA and strB are usually carried on pieces of DNA that can move among bacteria. The level of resistance (minimal inhibitory concentration) conferred to bacteria is greater from a chromosomal mutation, which renders the cell insensitive to the antibiotic, than from the production of a phosphotransferase enzyme that inactivates streptomycin (Shaw et al 1993). It should be noted, however, that the level of resistance conferred by the enzyme is still about five times greater than the concentration at which streptomycin is used in the field.
Streptomycin resistance in most isolates of E. amylovora has been attributed to chromosomal mutation because the majority of resistant isolates obtained from different locations do not harbor strA-strB. However, a quantitative survey to determine the relative prevalence of the resistance mechanisms in various geographical regions has not been undertaken. Molecular studies show that the chromosomal mechanism of resistance has arisen independently in E. amylovora multiple times (Chiou and Jones 1995b; McManus and Jones 1995).
The genes strA and strB usually reside on transposons carried on plasmids, mobile pieces of DNA that are generally not required for basic bacterial functions but that frequently carry antibiotic resistance genes. Transposon Tn5393 carries strA and strB in streptomycin-resistant strains of Erwinia, Pseudomonas, and Xanthomonas on plants (Chiou and Jones 1993; Sundin and Bender 1996a,b). StrA and strB have been identified in at least 17 environmental and clinical bacteria populating diverse niches (Sundin and Bender 1996a,b), and in most cases, the genes are on plasmids. In bacteria associated with animals, the plasmids carrying genes for streptomycin resistance are usually small (<10 kb) and able to exist in a broad range of bacterial species. These small plasmids do not have the genes necessary to move from cell to cell. However, they can be transferred with help from other bacterial gene products. In contrast, the resistance plasmids in plant-associated bacteria are usually large (>30 kb), relatively specific for certain bacterial host species, and able to accomplish their own cell-to-cell transfer. In fact, the most common resistance plasmid in E. amylovora is identical to a resistance plasmid found in P. agglomerans (formerly called Erwinia herbicola), a nonpathogenic bacterial species that is commonly found in apple orchards on trees, grasses, and weeds. This has led to the hypothesis that E. amylovora acquired the resistance genes strA and strB from its nonpathogenic cohorts (Chiou and Jones 1993). This could occur through plasmid transfer between two different bacteria living in close proximity on plant surfaces. An exception to the rule of relatively large resistance plasmids in plant-associated bacteria is the discovery of a small plasmid, similar to the broad host range plasmid RSF1010, in a few isolates of E. amylovora (Palmer et al 1997).
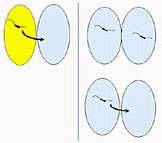 |
Hypothesized acquisition of streptomycin resistance genes (strA-strB) by Erwinia amylovora. Click on image for enlargement and more information. |
Regardless of the mechanisms, streptomycin resistance appears to be a stable trait in plant pathogenic bacteria. A survey of celery beds in Florida in the early 1990s detected widespread resistance to streptomycin among isolates of Pseudomonas cichorii, the causal agent of bacterial blight, even though streptomycin had not been used commercially for disease control since the late 1960s (Pohronezny et al 1994). Streptomycin-resistant isolates of E. amylovora still were detected in an orchard in California 10 years after applications of the antibiotic were halted (Moller et al 1980). Similarly, streptomycin-resistant strains of E. amylovora remain widespread in Washington state (Stockwell et al, unpublished data) even though usage of the antibiotic decreased dramatically in the 1990s after it was reported that 88% of the surveyed orchards harbored resistant strains (Loper et al 1991). Perhaps "compensatory mutations," a phenomenon recently described in streptomycin-resistant Escherichia coli (Levin et al 2000) accounts for the stability of streptomycin-resistant plant pathogen populations in the absence of selection. In this scenario, chromosomal mutations for antibiotic resistance may initially inflict a fitness burden to the bacterium. However, as the bacterium evolves in the absence of selection, it undergoes mutations that ameliorate the fitness costs. The end result of compensatory mutations is that the streptomycin-resistant strains persist.
Political Fallout
Does antibiotic use on plants pose a risk to human health? This question is the subject of contentious debate in the U.S., Europe, and elsewhere. One consumer advocacy group in the U.S. has gone so far as to call for a ban on antibiotics used as pesticides (Lieberman and Wootan 1998; Center for Science in the Public Interest http://www.cspinet.org/reports/abiotic.htm). Growers, however, defend their practice as being so limited in scope as to be inconsequential to the emergence of antibiotic resistance in hospitals and communities. With almost no research data to uphold either view, arguments are frequently based on circumstantial evidence and fueled by passion.
The greatest concern of those opposed to antibiotic use on plants is that spraying antibiotics in the open environment and over physically large expanses of land might increase the frequency of antibiotic resistance genes—not just streptomycin- and tetracycline-resistance genes but any other resistance genes that might be carried on the same plasmid—thereby increasing the risk of these genes finding their way into medically important bacteria. This would require the transfer of resistance genes from plant- and soil-borne bacteria to bacteria that reside on or in humans. The transfer would have to occur somewhere between the orchard and the human gut, in an environment sufficiently favorable to both the gene donor and the recipient. To date there is no circumstantial or experimental evidence for such a scenario. In addition to transfer of resistance genes from environmental bacteria to "human" bacteria, the genes would have to be functional in their new bacterial host in order to wreck havoc. We are aware of only one study in which this issue was addressed. Palmer and Jones (1999) found that streptomycin and tetracycline resistance genes were often carried on the same large plasmid in orchard bacteria, but when the plasmid was put into E. coli, the new host was resistant only to tetracycline and not to streptomycin or 10 other antibiotics.
Recently a Mexican company attempted to register a plant-grade gentamicin formulation with the U.S. EPA, primarily for fire blight control. The product is currently used on a variety of crops in Mexico and Central America and suppresses fire blight in field tests. Gentamicin, however, is a critical tool in human medicine, used alone or in combination with other drugs for treatment of various infections. In its evaluation of the product, EPA called on experts from CDC. The result of the consultation was that all antibiotic use on plants was called into question. In October 1999, the application for registration of gentamicin on crop plants in the United States was withdrawn.
At this point, streptomycin and oxytetracycline have been used on crop plants for the past 45 years and 25 years, respectively, without reports of adverse effects on humans. The efficacy of these silver bullets for control of plant diseases has been diminished in some areas due to the emergence of antibiotic resistant strains of pathogens, but they remain important tools for the management of some of the most serious plant diseases. The existing registrations of streptomycin and oxytetracycline with the EPA appear to be intact; however, it is extremely unlikely that any additional antibiotics will become available for use on plants.
References
Agrios, G.N. 1997. Plant Pathology, 4th Edition. Academic Press, San Diego.
Burr, T. J., J. L. Norelli, B. Katz, W. F. Wilcox, and S.A. Hoying. 1988. Streptomycin resistance of Pseudomonas syringae pv. papulans in apple orchards and its association with a conjugative plasmid. Phytopathology 78:410-413.
Calzolari, A., F. Finelli, and G.L Mazzoli. 1999. A severe unforeseen outbreak of fire blight in the Emilia-Romagna region. Acta Hort. 489:171-176.
Chiou, C.-S., and A. L. Jones. 1993. Nucleotide sequence analysis of a transposon (Tn5393) carrying streptomycin resistance genes in Erwinia amylovora and other gram-negative bacteria. J. Bacteriol. 175:732-740.
Chiou, C.-S., and A. L. Jones. 1995a. Expression and identification of the strA-strB gene pair from streptomycin-resistant Erwinia amylovora. Gene 152:47-51.
Chiou, C.-S., and A. L. Jones. 1995b. Molecular analysis of high-level streptomycin resistance in Erwinia amylovora. Phytopathology 85:324-328.
Coyier, D. L., and R. P. Covey. 1975. Tolerance of Erwinia amylovora to streptomycin sulfate in Oregon and Washington. Plant Dis. Rep. 59:849-852.
Cvjetkovic, B., E. Halupecki, and J. Spoljaric. 1999. The occurrence and control of fire blight in Croatia. Acta Hort. 489:71-73.
Goodman, R.N. 1959. The influence of antibiotics on plants and plant disease control. Pages 322-448. In: Antibiotics: their chemistry and non-medical uses. H.S. Goldberg, ed. D. van Nostrand and Company, Inc. Princeton, NJ.
Johnson, K. B., and V. O. Stockwell. 1998. Management of fire blight: A case study in microbial ecology. Annu. Rev. Phytopathol. 36:227-248.
Jones, A. L., J. L. Norelli, and G. R. Ehret. 1991. Detection of streptomycin-resistant Pseudomonas syringae pv. papulans in Michigan apple orchards. Plant Dis. 75:529-531.
Levin, B.R., V. Perrot, and N. Walker. 2000. Compensatory mutations, antibiotic resistance and the population genetics of adaptive evolution in bacteria. Genetics 154: 985-997.
Levy, S.B. 1992. The Antibiotic Paradox: How Miracle Drugs are Destroying the Miracle. Plenum Press, New York.
Lieberman, P. B., and M. G Wootan. 1998. Protecting the Crown Jewels of Medicine. Center for Science in the Public Interest Newsletter.
Lindow, S.E., G. McGourty, and R. Elkins. 1996. Interactions of antibiotics with Pseudomonas fluorescens strain A506 in the control of fire blight and frost injury of pear. Phytopathology 86:841-848.
Loper, J. E., M. D. Henkels, R. G. Roberts, G. G. Grove, M. J. Willett, and T. J. Smith. 1991. Evaluation of streptomycin, oxytetracycline, and copper resistance of Erwinia amylovora isolated from pear orchards in Washington State. Plant Dis. 75:287-290.
Manulis, S., D. Zutra, F. Kleitman, I. David, and M. Zilberstaine. 1999. Streptomycin resistance of Erwinia amylovora in Israel and occurrence of fire blight in pear orchards in the autumn. Acta Hort. 489:85-87.
Manulis, S., D. Zutra, F. Kleitman, O. Dror, M. Zilberstaine, and E. Shabi. 1998. Distribution of streptomycin-resistant strains of Erwinia amylovora in Israel and occurrence of blossom blight in the autumn. Phytoparasitica 26:223-230.
McManus, P. S., and A. L. Jones. 1994. Epidemiology and genetic analysis of streptomycin-resistant Erwinia amylovora from Michigan and evaluation of oxytetracycline for control. Phytopathology 84:627-633.
McManus, P. S., and A. L. Jones. 1995. Genetic fingerprinting of Erwinia amylovora strains isolated from tree-fruit crops and Rubus spp. Phytopathology 85:1547-1553.
Minsavage, G. V., B. I. Canteros, and R. E. Stall. 1990. Plasmid-mediated resistance to streptomycin in Xanthomonas campestris pv. vesicatoria. Phytopathology 80:719-723.
Moller, W. J., M. N. Schroth, and S. V. Thomson. 1981. The scenario of fire blight and streptomycin resistance. Plant Dis. 65:563-568.
National Agricultural Statistics Service. 1997. Agricultural Chemical Usage, 1995 Fruits Summary. No. 96172. U.S. Dept. Agriculture.
Palmer, E. L., and A.L. Jones. 1999. Distribution of tetracycline resistance genes and transposons among phylloplane bacteria in Michigan apple orchards. Appl. Environ. Microbiol. 65:4898-4907.
Palmer, E. L., B.L. Teviotdale, and A.L. Jones. 1997. A relative of the broad-host-range plasmid RSF1010 detected in Erwinia amylovora. Appl. Environ. Microbiol. 63:4604-4607.
Perombelon, M.C. and A. Kelman. 1980. Ecology of the soft rot erwinias. Ann. Rev. Phytopathol. 18:361-387.
Pohronezny, K., M. L. Sommerfeld, and R. N. Raid. 1994. Streptomycin resistance and copper tolerance among strains of Pseudomonas cichorii in celery seedbeds. Plant Dis. 78:150-153.
Scheck, H. J., J. W. Pscheidt, and L. W. Moore. 1996. Copper and streptomycin resistance in strains of Pseudomonas syringae from Pacific Northwest Nurseries. Plant Dis. 80:1034-1039.
Severin, V., F. Constantinescu, and F. Jianu. 1999. Appearance, expansion, and chemical control of fire blight (Erwinia amylovora) in Romania. Acta Hort. 489:79-84.
Shaffer, W. H., and R. N. Goodman. 1985. Appearance of streptomycin-resistant Erwinia amylovora in Missouri apple orchards. Phytopathology 75:1281.
Shaw, K. J., P. N. Rather, R. S. Hare, and G. H. Miller. 1993. Molecular genetics of aminoglycoside resistance genes and familial relationships of the aminoglycoside-modifying enzymes. Microbiol. Rev. 57:138-163.
Stall, R. E., and P. L. Thayer. 1962. Streptomycin resistance of the bacterial spot pathogen and control with streptomycin. Plant Dis. Rep. 46:389-392.
Stockwell, V.O., K. B. Johnson, and J.E. Loper. 1996a. Compatibility of bacterial antagonists of Erwinia amylovora with antibiotics used for fire blight control. Phytopathology 86:834-840.
Stockwell, V.O., D. Sugar, R. Spotts, K.B. Johnson, and J.E. Loper. 1996b. Recovery of streptomycin-resistant isolates of Erwinia amylovora from Oregon orchards. Phytopathology 86:S50 (Abst).
Sundin, G. W., and C. L. Bender. 1996a. Dissemination of the strA-strB streptomycin resistance genes among commensal and pathogenic bacteria from humans, animals, and plants. Mol. Ecol. 5:133-143.
Sundin, G. W., and C. L. Bender. 1996b. Molecular genetics and ecology of transposon-encoded streptomycin resistance in plant pathogenic bacteria. In: Molecular Genetics and Evolution of Pesticide Resistance. T. M. Brown, ed. American Chemical Society, Washington D.C.
Thomson, S. V., S. C. Gouk, J. L. Vanneste, C. N. Hale, and R. Clark. 1993. The presence of streptomycin resistant strains of Erwinia amylovora in New Zealand. Acta. Hortic. 338:223-225.
van der Zwet and S.V. Beer 1991. Fire Blight: Its nature, prevention, and control-A practical guide to integrated disease management. US Dep. Agric., Agric. Inform. Bull. No. 631, 34 pp.