Symptoms and signs
Fig. 1. Symptoms of center rot in onion foliage and bulb; A: White, bleached out, necrotic lesions on onion foliage; B: Necrotic lesions and bulb rot.
|
Disease symptoms on foliage and onion bulbs caused by four
Pantoea species are similar (Gitaitis
et al. 2002; Walcott
et al. 2002; Edens
et al. 2006; Stumpf
et al. 2018). Foliar symptoms produced by
Pantoea sp.
complsex start with water-soaked lesions spanning the length of the leaf blade, which gradually become blighted resulting in desiccation and collapse of the tissue. As the disease progresses in the plant, severe wilting and blighting of foliage can occur leading to complete foliage death (Gitaitis and Gay 1997). Bacterial movement from foliar tissue into bulb was demonstrated experimentally, where it was shown that
P. ananatis blade infection could lead to bulb decay (Carr
et al. 2013). Additionally, the authors observed that the specific bulb scale showing symptoms could be traced back to the corresponding infected blade (Fig. 1). Therefore, the authors concluded that protecting foliage of onion leaves can protect against bulb incidence of center rot.
Host Range and Geographic Distribution
Pantoea ananatis can cause disease on a broad range of plants, including many economically important crops, around the globe (Coutinho and Venter 2009). The first record of
P. ananatis causing plant disease was in 1928 where it was identified causing fruitlet rot on pineapple in Philippines (Serrano 1928). The “ananatis" species name derives from the first host of isolation, pineapple (Ananas comosus). P. ananatis can survive as an asymptomatic epiphyte or symptomatic endophyte on both dicots and monocots (Coutinho and Venter 2009). Gitaitis
et al. (2002) reported that
P. ananatis is widely distributed throughout Georgia on numerous weed species commonly found near onion production sites.
P. ananatis was first reported in the United States in Georgia in 1997. Since then, it has been identified in other onion growing regions of the United States including Colorado (Schwartz and Otto 2000), Michigan (Schwartz and Mohan 2008), New York (Carr
et al. 2010), and Pennsylvania (Pfeufer
et al. 2008). Additionally,
P. ananatis has been isolated from many economically important crops around the world including honeydew melon in Ecuador (Wells
et al. 1987), cantaloupe (Bruton
et al. 1991), onion (Gitaitis and Gay 1997), and sudangrass (Azad
et al. 2000) in the United States, eucalyptus in South Africa (Coutinho
et al. 2002), rice in Italy (Cortesi and Pizzatti 2007) and Russia (Egorova
et al. 2015), netted melon in Japan (Kido
et al. 2008), maize in Argentina (Alippi and Lopez 2010) and Poland (Krawczyk
et al. 2010), and sorghum in Brazil (Cota
et al. 2010).
P. agglomerans was first described as a causal agent for the center rot of onion in South Africa (1981). Later, the bacterium was identified as a causal agent of center rot in Georgia in 2006 (Hattingh and Walters. 1981; Edens
et al. 2006). This was the first report of the bacterium causing this disease in the United States (Edens
et al. 2006).
P. allii was identified as the third member in the
Pantoea species complex causing center rot of onion in Georgia (Brady
et al., 2011). Recently,
P. stewartii subsp.
indologenes was included as the fourth member in the center rot complex of onion along with
P. ananatis,
P. agglomerans and
P. allii (Stumpf
et al., 2018).
Pathogen biology and molecular identification
Pantoea ananatis is a Gram-negative, rod-shaped, facultative anaerobe similar to other members of the
Enterobacteriales (Kim
et al. 2012; Gitaitis and Gay 1997). Colonies are yellow pigmented and the cells utilize glucose in an oxidative and fermentative manner. The bacterium tests positive for the following biochemical assays: β-D-galactosidase and catalase, utilization of citrate, and production of acetoin, and indole.
P. ananatis tests negative for ornithine decarboxylase, lysine decarboxylase, urease, and oxidase (Gitaitis and Gay 1997). Strains of other closely related Pantoea spp., namely
P. agglomerans, P. alli, and
P. stewartii subsp.
indologenes have been isolated that can cause onion center rot symptoms. Identifying the causal agent of center rot infection is important in implementing an effective management strategy, as the epidemiology among the four
Pantoea species may be different. In general,
P. ananatis and
P. alli can be differentiated from
P. agglomerans as they produce a positive reaction for indole production and a negative reaction for phenylalanine deaminase and nitrate reductase enzymes.
P. ananatis can be differentiated from
P. allii based on acid production from amygdalin.
P. allii produces acid from amygdalin, while
P. ananatis does not (Brady
et al. 2011). However,
P. stewartii subsp.
indologenes cannot be differentiated biochemically from
P. ananatis nor
P. alli.
The sequencing of 16S rRNA is considered to be the standard method for elucidating phylogenetic relationships among bacterial pathogens. In
Pantoea spp., the analysis of the 16S rRNA gene revealed a close phylogenetic relationship among
P. agglomerans, P. allii, and P. ananatis, as a result this test is unreliable to distinguish these species. (Hauben
et al. 1998; Naum
et al. 2008). Gevers
et al. (2005) also contradicted the dependability of the 16S rRNA gene as a reliable phylogenetic marker due to the occurrence of potential recombination or horizontal gene transfer events in bacteria that may distort the actual relationship. A more reliable sequence-based method for genotyping strains is multilocus sequence analysis (MLSA). This approach uses the sequences of five to seven housekeeping genes to define sequence profiles of similar strains. Briefly, the 16S rRNA gene sequence may reliably identify strains to the genus level, but not to the correct species level. Sequencing housekeeping genes is the preferred method to differentiate closely related
Pantoea species (Gevers
et al. 2005).
Accurate identification is fundamental to understand pathogen spread, epidemiology, and management of bacterial pathogens. The current methods of serological techniques and 16S rRNA sequencing may fail to recognize the introduction of exotic strains or the development and spread of strains with enhanced virulence (Barak and Gilberston 2003). Delétoile
et al. (2009) characterized 36
Pantoea strains, including 28 strains from diverse origins (hosts, geographical locations, and ecological niches) initially identified as
P. agglomerans using MLSA based on six protein-coding genes (fusA,
gyrB,
leuS,
pyrG,
rplB, and
rpoB), and using biochemical and antimicrobial susceptibility assays. Phylogenetic analysis and subsequent comparison with other Enterobacteriales species revealed that the genus
Pantoea is highly diverse. All 20
P. agglomerans strains could be distinguished by multilocus sequence typing, indicating a high power of discrimination by MLSA for strain typing and population structure in this species (Delétoile
et al. 2009). The authors also found that biochemical characteristics such as fosfomycin resistance and utilization of d-tartrate could differentiate
P. agglomerans from other
Pantoea species. Furthermore,
repA gene was detected, known to be only associated with pathogenicity in plants, was also present in all clinical strains (Delétoile
et al. 2009). Therefore, it was concluded that clinical and plant-associated strains do not form distinct populations. Altogether, the study indicated that MLSA is a powerful tool for
Pantoea species delineation and identification and for strain tracking (Delétoile
et al. 2009).
Of the six housekeeping genes previously described, an in-depth comparison of tree topologies derived from single genes indicates that the tree topology for the gene
leuS, that encodes leucyl-tRNA synthetase, is similar to the topology produced from the concatenation of previously mentioned genes (Tambong
et al. 2014). This gene may be a strong phylogenetic marker for
Pantoea due to low recombination frequency and stable polymorphic sites within the coding sequence (Tambong
et al. 2014).
Three primer sets are available for the detection of
P. ananatis based on the 16S-23S ribosomal internal transcribed spacer (ITS) region. Although the primers were designed from the 16S-23S ITS region of
P. ananatis, they cross-reacted with
P. allii and
P. stewartii subsp. indologenes (Carr
et al. 2010; Figueiredo and Paccola-Meirelles 2012; Gitaitis
et al. 2002). Asselin
et al. (2016) developed PCR primers for detection of
P. ananatis,
Burkholderia sp., and
Enterobacter sp. from onion which is specific to
P. ananatis and, to date, they have not cross-reacted with other closely related
Pantoea species. PCR primers specific for
P. agglomerans are not available and the detection relies on 16S rRNA sequencing and MLSA. Two sets of
P. stewartii subsp.
indologenes specific primers are available based on
recA and
galE protein-coding genes that are routinely used for detection (Gehring
et al. 2014).
Disease cycle and epidemiology
The life cycle of
P. ananatis is complex, as bacteria can overseason to infect onions in a number of different ways (Fig. 2). Like many bacterial pathogens,
P. ananatis can be seed-borne with infested seed serving as a survival mechanism as well as a means of dissemination (Walcott
et al. 2002). Walcott
et al. demonstrated that
P. ananatis can be both naturally seed-borne and seed-transmitted in onion. The authors collected seeds from umbels of onion plants with no obvious symptoms. Using an immunomagnetic separation and polymerase chain reaction (IMS-PCR) assay with species-specific primers,
P. ananatis was detected in naturally infested onion seed. Although
P. ananatis did not affect germination rates, the pathogen did cause necrosis on germinating seedlings. The significance of the bacterium's ability to colonize seed is uncertain, as most onion seed production sites are located in arid climates. Nonetheless, understanding
P. ananatis' ability to infest seeds and deciphering its mechanism of seedling transmission are critical to understand center rot epidemiology (Walcott
et al. 2002).
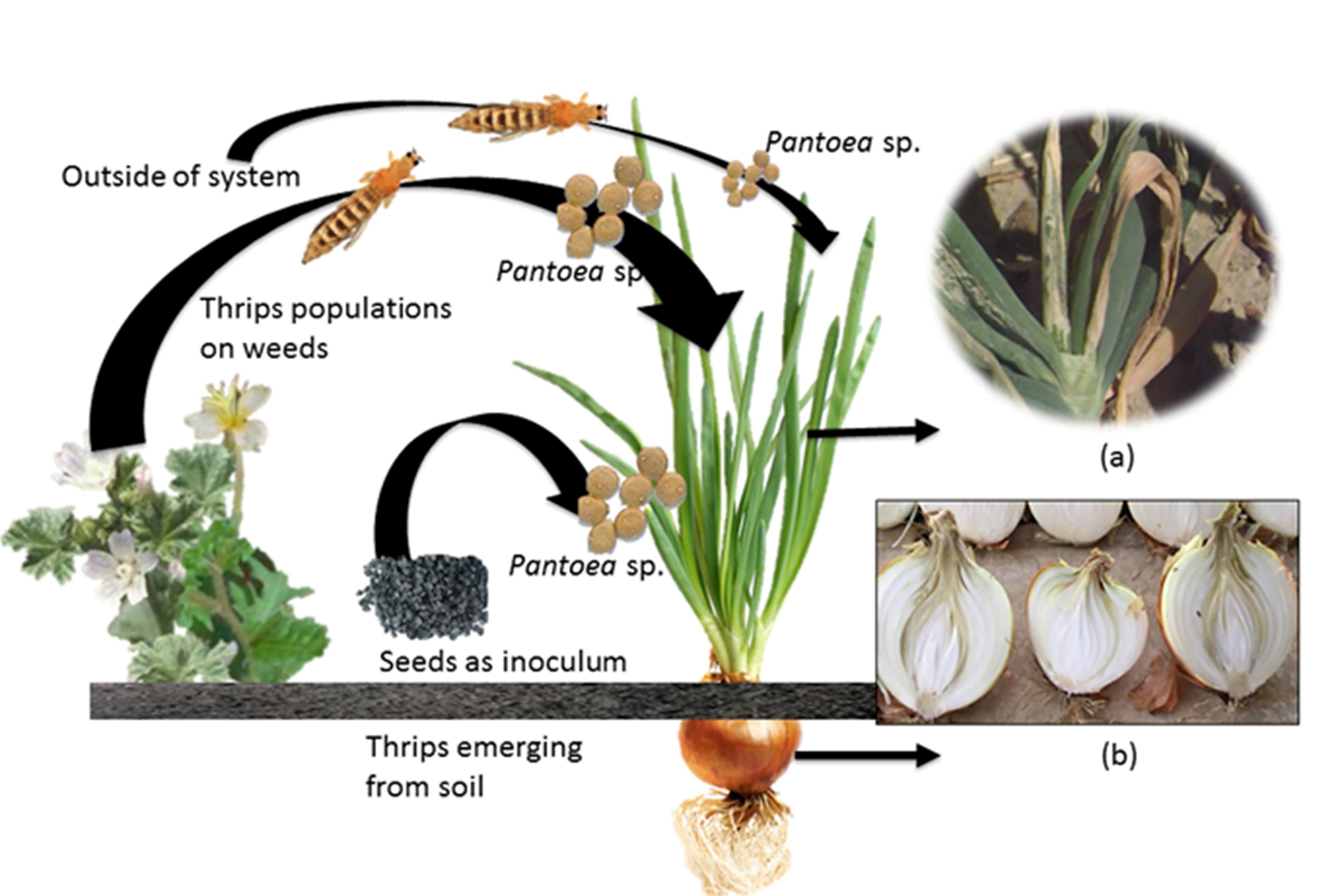 Fig. 2. Center rot-onion pathosystem: Multiple sources of inoculum (seed, weed and thrips) contribute to center rot epidemic in field; A: foliar symptom resulting in reduced yield (pre-harvest) and B: bulb rot in storage resulting in post-harvest losses
|
Although
P. ananatis can be seedborne, the proposed primary mode of transmission is by two insect vectors (Gitaitis
et al. 2003; Wells
et al. 2002). Two species of thrips, tobacco thrips (Frankliniella fusca (Hinds)) and onion thrips (Thrips tabaci), have the ability to transiently acquire and transmit
P. ananatis and
P. agglomerans (Gitaitis
et al. 2003; Dutta
et al. 2014), which are prevalent in the onion-producing region of Georgia specifically Vidalia onion region. The Vidalia onion region comprises of 21 counties in the southeastern part of Georgia, where specialty sweet Vidalia onions are grown. Immunolabeling with
P. ananatis-specific antibody indicated that
P. ananatis was localized in the gut of
T. tabaci (Fig. 3). Further investigation revealed that
P. ananatis could not be detected in salivary secretions of
T. tabaci despite feeding on a contaminated food source, but rather detected from fecal rinsates. The authors proposed that simultaneous feeding and defecating may facilitate bacterial ingress through feeding wounds from feces. The bacterium can persist in a non-circulative manner in the gut of thrips for 128 h, allowing the vector to infect plants over an extended period of time (Dutta
et al. 2014).
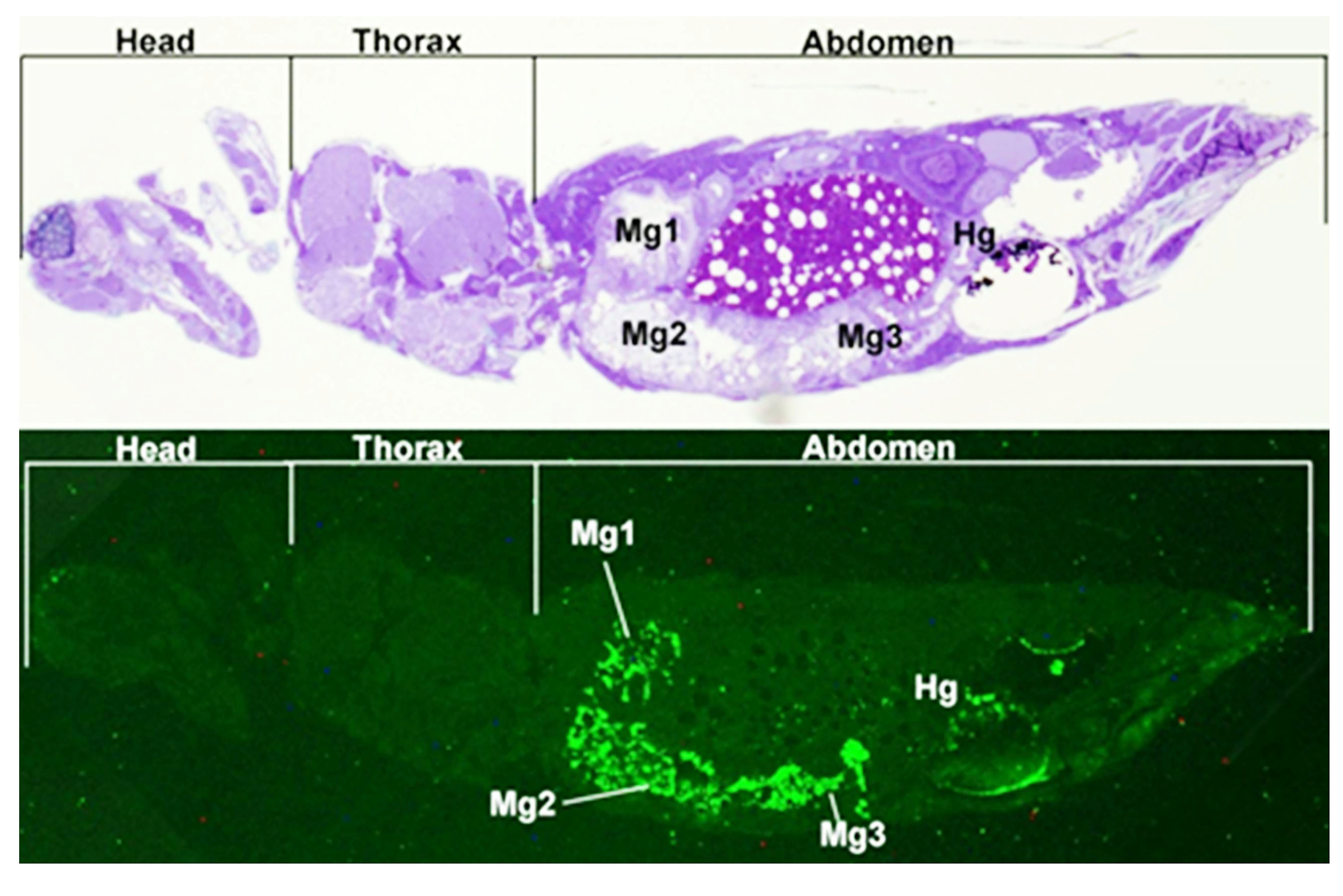 Fig. 3. Immunolocalization of
Pantoea ananatis in adult Thrips tabaci. (Top) Longitudinal section stained with toluidine blue of
Thrips tabaci that had fed on epiphytic populations of
Pantoea ananatis; (Bottom) Same section as above using fluorescent microscopy to highlight
P. ananatis cells labeled with a fluorescent antibody. Mg1 = midgut 1, Mg2 = midgut 2, Mg3 = midgut 3, and Hg = hindgut.
|
Further studies were conducted to investigate interactions of
F. fusca and
P. ananatis. Both adults and larvae of
F. fusca were able to acquire the bacterium, which further persisted transstadially. Moreover, both thrips' life stages were able to acquire
P. ananatis within one hour of access to a contaminated food source, and by 48 h approximately 70% of thrips acquired the pathogen (Dutta
et al. 2016). Mechanical inoculation of fecal rinsates on onion showed
P. ananatis was able to infect tissue, while sucrose solution potentially containing salivary secretions were not able to cause symptoms suggesting the bacterium is not circulative. These observations support the hypothesis that
P. ananatis infection occurs from defecation near wounding sites (Dutta
et al. 2016).
P. ananatis can survive epiphytically and endophytically on a wide range of hosts. These alternative hosts can serve as a source of inoculum in fields where susceptible crops are grown. In Georgia alone, 25 weed species, including carpetweed (Mollugo verticillata), common ragweed (Ambrosia artemisiifolia), crabgrass (Digitaria sanguinalis), common cocklebur (Xanthium pensylvanicum), curly dock (Rumex crispus), Florida pusley (Richardia scabra), sicklepod (Cassia obtusifolia), stinkweed (Thlaspi arvense), Texas panicum (Panicum texanum), vaseygrass (Paspalum urvillei), wild radish (Brassica spp.), yellow nutsedge (Cyperus esculentus) and other multiple crop plants were found to harbor
P. ananatis populations asymptomatically (Gitaitis
et al. 2002). Many of these weeds were found on or nearby onion production fields providing a local inoculum source (Gitaitis
et al. 2002).
Of the weeds
P. ananatis was found to survive consistently on Florida pusley (R. scabra) (Gitaitis
et al. 2002). Dutta
et al. (2017) investigated how
P. ananatis survived epiphytically under different temperature and moisture regimes that mimicked conditions in the Vidalia onion region from March to May.
P. ananatis populations survived significantly better at 21.1°C than 15.5°C under different moisture regimes (12 h wet/12 h dry; 12 h dry/12 h wet; continuous wet) (Dutta
et al. 2017). Bacterial colonies were recovered from leaves up to 72 hours post-inoculation. However, epiphytic survival was not observed under a continuous dry regime.
P. ananatis epiphytic populations survived longer on
R. scabra leaves with prolonged leaf wetness at a temperature common from March to May in the Vidalia onion region. Therefore, weeds near onion production sites may harbor epiphytic bacterial populations for prolonged periods when onions are nearing maturity (Dutta
et al. 2017).
Disease management
Onion cultivars resistant to
Pantoea sp. are not commercially available. Use of certified onion seeds is encouraged to avoid introduction of
Pantoea sp. inoculum in the production field. Planting early maturing or mid-maturing onion varieties are often recommended for growers. Late maturing varieties provide a larger window for infection and a potential epidemic to occur, which are favored by thrips pressure, hot and humid conditions, and lack of effective bactericides. These conditions are more prevalent in the southeastern United States compared to other onion growing regions of country. Early maturating varieties are able to avoid conditions that are suitable for bacterial disease development and
P. ananatis infection. The overhead irrigation should be avoided as it promotes bacterial spread compared with sub-surface or drip-irrigation. Controlling thrips population can be an effective management strategy to reduce center rot incidence as these vectors play an important role in bacterial transmission (Dutta et al., 2014, 2016). An integrated approach that targets sources of inoculum to counter bacterial spread is desirable for center rot management.
Center rot management in onion fields relies heavily on copper applications mixed with an ethylenebisdithiocarbamate fungicide (EBDC), such as mancozeb, which growers may apply weekly as a protectant (Pfeufer
et al. 2015). In addition, researchers found
P. ananatis strains to be copper-tolerant indicating overuse and potential risk of insensitivity to this chemistry (Nischwitz
et al. 2007). It has been suggested that in other
Enterobacteriales members such as
Escherichia coli and
Enterococcus hirae, tolerance to copper is mediated by an efflux system, which actively expels the copper ions out of bacterial cell (Weiner et al., 1999). Another mechanism suggests the role of 'cop operon' involving periplasmic, inner and outer membrane protein coding genes that sequester copper ion in the periplasm and outer membrane (Cooksey 1990, 1994). However, no such mechanism has yet been confirmed in
P. ananatis. Repeated applications of copper sprays during susceptible growth stages can be effective only to a limited extent and does not offer a robust solution to the problem. Perhaps the inefficacy of these sprays could be due to thrips preference to colonize certain parts of the onion plant, e.g. the basal meristems (neck region). Applications of copper and insecticide may not come in contact with the basal meristem leaving it susceptible to infection (Gitaitis
et al. 2003). A cultivar bred with a wide angle of divergence of the innermost leaves, and leaf sheaths elongating beyond the older leaves may prevent thrips from colonizing this area (Boivin
et al. 1995; Brewster 1987, 2008).
The implementation of successful weed management strategies are important in reducing
P. ananatis inoculum in the field. By reducing weeds, growers can potentially reduce initital inoculum. For example, effective weed management has been observed to reduce the spread of
Pseudomonas viridiflava in onion farmscapes located in Georgia (Gitaitis
et al. 2004).
Genome architecture of
P. ananatis
The
P. ananatis genome ranges from 4.3 Mb (P. ananatis S6) to 5.25 Mb (P. ananatis DAR 76143) suggesting substantial strain-to-strain variation in gene content.
P. ananatis genomes are predicted to encode over 4000 genes (Stuart
et al. 2017). The genomes of 32 publicly released
P. ananatis strains consist of one large circular chromosome and the large
Pantoea plasmid (LPP-1) in addition to variable accessory plasmids (Ismail
et al. 2014). The LPP-1 plasmid ranges in size from 280.8 to 352.8 Kb and typically encodes 200-300 predicted genes (Choi
et al. 2012; Weller-Stuart
et al. 2014).
P. ananatis genome sequencing efforts have focused on phytopathogenic strains known to infect plant hosts including pineapple (Adam
et al. 2014), rice (Choi
et al. 2012), eucalyptus (De Maayer
et al. 2012), cotton and maize (Medrano and Bell, 2015, Weller-Stuart
et al. 2014) as well as saprophytic and plant growth promoting strains (De Maayer
et al. 2014). A collection of 10 Georgia
P. ananatis isolates including six onion pathogenic and four non-pathogenic strains were sequenced to identify genes related to onion pathogenicity in
P. ananatis (Stice
et al. 2018).
Variation in pathogenicity and aggressiveness has been linked to variations in the mobile accessory genome. The LPP-1 plasmid has been proposed to make major contributions to
P. ananatis genomic plasticity and phenotypic adaptability (De Maayer
et al. 2012).
P. ananatis LPP-1 is derived from a plasmid ancestral to the
Pantoea genus and has undergone extensive diversification in terms of its genetic composition. Several phage-associated regions and mobile genetic elements have been accumulated in the plasmid genome during the course of its evolution. Many of the plasmid encoded genes such as antibiotic resistance, iron and nitrogen assimilation, and host-microbe interaction determinants are proposed to contribute to the phenotypic diversity of this bacterium (De Maayer
et al. 2012). An integrative and conjugative element (ICEPan) has also been proposed to contribute to phenotypic diversity. The ICEPan element encodes for proteins involved in antibiosis and stress responses which may contribute to the occupation of diverse niches (De Maayer
et al. 2015). A recent study by Stice
et al. (2018) identified 57 genes in four contiguous blocks that correlated with the ability of
P. ananatis to cause both foliar and bulb symptoms in onion. These regions were named Onion Virulence Regions A-D (OVRA-D) and are carried on an accessory plasmid. The OVR loci contain genes annotated as involved in thiol and redox regulation, sugar transporters as well as pectate lyase and rhamnogalacturonase cell wall degrading enzymes.
Virulence Factors
P. ananatis is unusual among phytopathogenic bacteria in that it does not encode a virulence-associated Type III secretion system (T3SS), a common pathogenicity determinant among other bacterial plant pathogens including
P. stewartii subsp.
stewartii, nor a Type II secretion system (T2SS) used by many soft rot pathogens to secrete cell wall degrading enzymes (Stice
et al. 2018).
P. ananatis encodes several Type VI secretion system (T6SS) loci some of which have been shown to contribute to inter-bacterial competition and symptom development in onion leaves (Shyntum
et al. 2014). Other factors identified with critical roles in onion center rot include the EanI/EanR N-acyl homoserine lactone quorum regulation system (Morohoshi
et al. 2007) and flagellar swimming motility (Weller-Stuart
et al. 2017). Twitching motility was also observed to make a modest contribution to disease (Weller-Stuart
et al. 2017). In addition,
P. ananatis encodes the gene synthesis cluster for 'stewartan' exopolysaccharide production, a critical virulence factor for
P. stewartii subsp.
stewartii (Stice
et al. 2018). Interestingly, both flagellar gene expression and EPS production are quorum regulated in
P. ananatis (Morohoshi
et al. 2007; Sibanda
et al. 2018). A recent study by Asselin et al. (2018) identified a chromosomal cluster of 16 genes that is strongly associated with virulence of
P. ananatis in onion. This gene cluster, called HiVir, has a reduced GC content, relative to the balance of the genome, indicating its potential acquisition via horizontal gene transfer (Fig. 4). Inactivation of the Major Facilitator Family exporter gene (HiVir gene K) was associated with reduced lesion length on onion leaves. The HiVir cluster also contains
pepM, a gene annotated as phosphoenolpyruvate phosphomutase (Fig. 4). Deletion of the
pepM gene (HiVir gene C) is associated with the loss of symptom production in both onion leaves and bulbs, as well as a large reduction in bacterial multiplication.
PepM is commonly required for the initial step in the biosynthetic pathways for phosphonates and phosphinates. The authors proposed that the HiVir cluster is required for the production of an as of yet unidentified phosphonate or phosphinate secondary metabolite that plays a major role in the pathogenicity of P. ananatis in onion (Asselin et al. 2018).
Fig 4. Diagram of the proposed HiVir gene cluster region from
P. ananatis LMG 20103(PANA_3292- PANA_3277). Onion plants 5 days after inoculation with
P. ananatis OC5a Rpr wild type (WT) and derivatives. A, WT; B, ΔpepM mutant; C, ΔpepM complemented in trans; D, ΔpepM harboring empty vector. (Reproduced from Asselin et al. 2018)
|
Significance
Center rot has emerged as a chronic problem in a number of onion growing regions in the United States, and has been responsible for significant pre- and post-harvest losses in yield and quality. Like many bacterial pathogens,
P. ananatis can be seedborne with infested seed serving as a survival mechanism as well as a means of dissemination (Walcott
et al. 2003). Also, as with many bacteria, these pathogens can survive on several weed species (Gitaitis
et al. 2002). Finally,
P. ananatis can be transmitted by tobacco and onion thrips making it the only plant pathogenic bacterial pathogen currently known to be thrips transmitted (Gitaitis
et al. 2003; Dutta
et al. 2014, 2016). Host resistance against these pathogens has not yet been characterized and onion varieties with resistance/tolerance to
Pantoea spp. has not been identified. The multiple sources of inoculum (seed, weeds, and insects) and a lack of genetic resistance to P. ananatis requires evaluation of mitigation strategies for each inoculum source and ultimately a comprehensive, resilient disease management strategy against this pathogen. Breeding for host resistance will be critical for future management of this disease. For disease management and resistance breeding to be effective, an understanding of virulence mechanisms and the pathogen population structure is necessary. Representative strain(s) of taxa within the pathogen population should be considered when screening germplasms, because a diverse pathogen population may overcome resistance rather quickly, or the host may not express complete resistance. On the contrary, a population in a specific geographic region lacking diversity may be less challenging to breed resistance against
P. ananatis. Hence, the future research should focus on understanding virulence mechanisms and bacterial population structure and strategies to develop advanced breeding lines that are resistant to
Pantoea sp.
Literature Cited
Adam, Z., Tambong, J. T., Lewis, C. T., Levesque, C. A., Chen, W., Bromfield, E. S. P., Khan, I.U.H. and Xu, R. 2014. Draft genome sequence of
Pantoea ananatis strain LMG 2665T, a bacterial pathogen of pineapple fruitlets. Genome Announc. doi: 10.1128/genomeA.00489-14.
Asselin, J. A. E., Bonasera, J. M. and Beer, S. V. 2016. PCR Primers for Detection of
Pantoea ananatis,
Burkholderia spp., and
Enterobacter sp. from onion. Plant Dis. 100:836-846.
Asselin, J. A. E., Bonasera, J. M. and Beer, S. V. 2018. Center rot of onion (Allium cepa) caused by
Pantoea ananatis requires PepM, a predicted phosphonate-related gene. Mol. Plant-Micr. Interact. 31:1291-1300.
Alippi, A. M., and López, A. C. 2010. First report of leaf spot disease of maize caused by
Pantoea ananatis in Argentina. Plant Dis. 94:487.
Azad, H. R., Holmes, and G. J., Cooksey, D. A. 2000. A new leaf blotch disease of sudangrass caused by
Pantoea ananas and
Pantoea stewartii. Plant Dis. 84:973-979.
Barak, J. D., and Gilbertson, R. L. 2003. Genetic diversity of
Xanthomonas campestris pv.
vitians, the causal agent of bacterial leafspot of lettuce. Phytopathology 93:596-603.
Boivin, G., Fournier, F., and Stewart, R. K. 1995. Effect of
Thrips tabaci (Thysanoptera: Thripidae) on yellow onion yields and economic thresholds for its management. J Econ. Entomol. 88:1401.
Brady, C. L., Goszczynska, T., and Venter, S. N. 2011.
Pantoea allii sp. nov., isolated from onion plants and seed. Int. J. Syst. Evol. Biol. 61:932-937.
Brewster, J. L. 1987. The effect of temperature on the rate of sprout growth and development within stored onion bulbs. Ann. Appl. Biol. 111:463.
Brewster, J. L. 2008. The structure of edible Alliums. Pages 27-47 in: Onions and Other Vegetable Alliums. 2nd ed. CABI, Cambridge.
Bruton, B. D., Wells, J. M., Lester, G. E., and Patterson, C. L. 1991. Pathogenicity and characterization of
Erwinia ananas causing a postharvest disease of cantaloupe fruit. Plant Dis. 75:180-183.
Carr, E. A., Bonasera, J. M., Zaid, A. M., Lorbeer, J. W., and Beer, S. V. 2010. First report of bulb disease of onion caused by
Pantoea ananatis in New York. Plant Dis. 94:916
Carr, E. A., Zaid, A. M., Bonasera, J. M., Lorbeer, J. W., and Beer, S. V. 2013. Infection of onion leaves by
Pantoea ananatis leads to bulb infection. Plant Dis. 97:1524-1528.
Choi, O., Lim, J. Y., Seo, Y. S., Hwang, I. and Kim, J. 2012. Complete genome sequence of the rice pathogen
Pantoea ananatis strain PA13. J. Bacteriol. 19:531.
Cortesi, P., and Pizzatti, C. 2007. Palea browning, a new disease of rice in Italy caused by
Pantoea ananatis. J. Plant Pathol. 89:S76.
Cooksey, D. A. 1990. Genetics of bactericide resistance in plant pathogenic bacteria. Annu. Rev. Phytopathol. 28:201-219. 14.
Cooksey, D. A. 1994. Molecular mechanisms of copper resistance and accumulation in bacteria. FEMS Microbiol. Rev. 14:381-386.
Cota, L. V., Costa, R. V., Silva, D. D., Parreira, D. F., Lana, U. G. P., and Casela, C. R. 2010. First report of pathogenicity of
Pantoea ananatis in sorghum (Sorghum bicolor) in Brazil. Australas. Plant Dis. 5:120-122.
Coutinho, T. A., Preisig, O., and Mergaert, J. 2002. Bacterial blight and dieback of Eucalyptus species, hybrids, and clones in South Africa. Plant Dis. 86: 20-25.
Coutinho, T. A., and Venter, S. N. 2009.
Pantoea ananatis: an unconventional plant pathogen. Mol. Plant Pathol. 10:325-335.
Delétoile, A., Decre, D., Courant, S. 2009. Phylogeny and identification of
Pantoea species and typing of Pantoea agglomerans strains by multilocus gene sequencing. J. Clin. Microbiol. 47:300-310.
De Maayer, P., Venter, S. N., Kamber, T., Duffy, B., Coutinho, T. A. and Smits, T. H. M. 2011. Comparative genomics of the type VI secretion systems of Pantoeaand Erwinia species reveals the presence of putative effector islands that may betranslocated by the VgrG and Hcp proteins. BMC Genomics 12:576-590.
De Maayer, P., Chan, W. Y., Blom, J., Venter, S. N., Duffy, B., Smits, T. H. and Coutinho, T. A. 2012 The large universal Pantoea plasmid LPP-1 plays a major role in biological and ecological diversification. BMC Genomics 64-13-625.
De Maayer, P., Chan, W. Y., Martin, D. A. J., Blom, J., Venter, S. N., Duffy, B., Cowan, D. A., Smits, T. H. and Coutinho, T. A. 2015. Integrative conjugative elements of the ICEPan family play a potential role in
Pantoea ananatis ecological diversification and antibiosis. Front. Microbiol. doi: 10.3389/fmicb.2015.00576.
Dutta, B., Barman, A. K., Srinivasan, R., Avci, U., Ullman, D. E., Langston, D. B., and Gitaitis, R. D. 2014. Transmission of
Pantoea ananatis and
P. agglomerans, causal agents of center rot of onion (Allium cepa), by onion thrips (Thrips tabaci) through feces. Phytopathology 104:812-819.
Dutta, B., Gitaitis, R., Barman, A., Avci, U., Marasigan, K., and Srinivasan, R. 2016. Interactions between
Frankliniella fusca and
Pantoea ananatis in the center rot epidemic of onion (Allium cepa). Phytopathology. 106:956-962.
Dutta, B., Anderson, F., Smith, S., and Gitaitis, R. 2017. Epiphytic survival of
Pantoea ananatis on
Richardia scabra L. in Georgia. Plant Dis. 101:613-618.
Egorova, M., Mazurin, E., Ignatov, A. N. 2015. First report of
Pantoea ananatis causing grain discolouration and leaf blight of rice in Russia. New Dis. Rep. 32:21.
Figueiredo, J. E. F. and Paccola-Meirelles, L. D. 2012. Simple, rapid and accurate PCR-based detection of
Pantoea ananatis in maize, sorghum and
Digitaria sp. J. Plant Pathol. 94:663-667.
Gevers, D., Cohan, F. M., and Lawrence, J. G. 2005. Re-evaluating prokaryotic species. Nat. Rev. Microbiol. 3:733-739.
Gehring, I., Wensing, A., Gernold, M., Wiedemann, W., Coplin, D. L. and Geider, K. 2014. Molecular differentiation of
Pantoea stewartii subsp.
indologenes from subspecies
stewartii and identification of new isolates from maize seeds. J. Appl. Microbiol. 116:1553-1562.
Gitaitis, R. D., and Gay, J. D. 1997. First report of a leaf blight, seed stalk rot, and bulb decay of onion by
Pantoea ananas in Georgia. Plant Dis. 81:1096.
Gitaitis, R. D., Walcott, R. R., Culpepper, S., Sanders, H., Zolobowska, L., and Langston, D. 2002. Recovery of
Pantoea ananatis, causal agent of center rot of onion, from weeds and crops in Georgia, USA. Crop Prot. 21:983-989.
Gitaitis, R. D., Walcott, R. R., Wells, M. L., Diaz-Perez, J. C., and Sanders, F. H. 2003. Transmission of
Pantoea ananatis, causal agent of center rot of onion, by tobacco thrips,
Frankliniella fusca. Plant Dis. 87:675-678.
Gitaitis, R. D., Walcott, R. R., Sanders, H. F., Zolobowska, L., and Diaz-Perez, J. C. 2004. Effects of mulch and irrigation system on sweet onion. II. The epidemiology of center rot. J. Am. Soc. Hortic. Sci. 129:225-230.
Gonçalves, R. M., Meirelles, W. F., Figueiredo, J. E. F., Balbi-Peña, M. I., and Paccola-Meirelles, L. D. 2015.
Digitaria horizontalis and
D. insularis as alternative hosts for
Pantoea ananatis in Brazilian maize fields. J. Plant Pathol. 97:177-181.
Griffiths, G., Trueman, L., Crowther, T., Thomas, B., and Smith, B. 2002. Onions—A Global benefit to health. Phytother. Res. 16:603-615.
Hauben, L., Moore, E. R., and Vauterin, L. 1998. Phylogenetic position of phytopathogens within the
Enterobacteriaceae. Syst. Appl. Microbiol. 21:384-397.
Ismail, E., Blom, J., Bultreys, A., Ivanovic, M., Obradovic, A., van Doorn, J.,Bergsma-Vlami, M., Maes, M., W illems, A., Duffy, B., Stockwell, V. O., Smits,T. H. M. and Pulawska, J. 2014. A novel plasmid pEA68 of
Erwinia amylovora and the description of a new family of plasmids. Arch. Microbiol. 196:891-899.
Jones, H. A., and Mann, L. K. 1963. Morphology and development. Pages 54-56 in: Onions and Their Allies: Botany, Cultivation, and Utilization. L. Hill, London.
Kido, K., Adachi, R., and Hasegawa, M. 2008. Internal fruit rot of netted melon caused by
Pantoea ananatis (=
Erwinia ananas) in Japan. J. Gen. Plant Pathol. 74:302-312.
Kim, J., Choi, O., and Kim, T. S. 2012. An outbreak of onion center rot caused by
Pantoea ananatis in Korea. Plant Dis. 96:1576.
Krawczyk, K., Kamasa, J., Zwolinska, A., and Pospieszny, H. 2010. First report of
Pantoea ananatis associated with leaf spot disease of maize in Poland. J. Plant Pathol. 92:807-811.
Martín-Sanz, A., Pérez de la Vega, M., Murillo, J., and Caminero, C. 2013. Strains of
Pseudomonas syringae pv.
syringae from pea are phylogenetically and pathogenically diverse. Phytopathology 103:673-681.
Medrano, E. G. and Bell, A. A. 2015. Genome sequence of
Pantoea ananatis strain CFH 7-1, which is associated with a vector-borne cotton fruit disease. Genome Announc. doi: 10.1128/genomeA.01029-15.
Mehta, A., Leite, R. J., and Rosato, Y. B. 2001. Assessment of the genetic diversity of
Xylella fastidiosa isolated from citrus in Brazil by PCR-RFLP of the 16S rDNA and 16S-23S intergenic spacer and rep-PCR fingerprinting. Antonie van Leeuwenhoek 79:53-59.
Melanson, R. A., Sanderlin, R. S., McTaggart, A. R., and Ham, J. H. 2012. A systematic study reveals that
Xylella fastidiosa strains from pecan are part of
X. fastidiosa subsp.
multiplex. Plant Dis. 96:1123-1134.
Morohoshi, T., Nakamura, Y., Yamazaki, G., Ishida, A., Kato, N., and Ikeda, T. 2007. The plant pathogen
Pantoea ananatis produces N-Acylhomoserine lactone and causes Center rot disease of onion by quorum sensing. J. Bacteriol. 189:8333-8338.
Nadarasah, G., and Stavrinides, J. 2014. Quantitative evaluation of the host-colonizing capabilities of the enteric bacterium
Pantoea using plant and insect hosts. Microbiology 160:602-615.
Naum, M., Brown, E. W., and Mason-Gamer, R. J. 2008. Is 16S rDNA a reliable phylogenetic marker to characterize relationships below the family level in the
Enterobacteriaceae? J. Mol. Evol. 66:630-642.
Nischwitz, C., Gitaitis, R., and Sanders, H. 2007. Use of fatty acid methyl ester profiles to compare copper-tolerant and copper-sensitive strains of
Pantoea ananatis. Phytopathology 97:1298-1304.
Pfeufer, E., Hoepting, C., and Gugino, B. 2015. Advances in managing onion bacterial diseases in the northeastern US. Onion World 31:22-27.
Riley, D. G., Sparks, A. N. Jr., and Chitturi, A. 2014. Current status of thrips (Thysanoptera: Thripidae) in Vidalia onions in Georgia. Fla. Entomol. 97:355-361.
Schwartz, H. F., and Mohan, S. K. 2008. Center Rot. Pages 64-66 in: Compendium of onion and garlic diseases and pests. APS Press, St. Paul, MN.
Schwartz, H. F., and Otto, K. 2000. First report of a leaf blight and bulb decay of onion by
Pantoea ananitas in Colorado. Plant Dis. 84:808.
Sibanda, S., Kwenda, S., Tanui, C. K., Shyntum, D. Y., Coutinho T. A., Moleleki, L. N. 2018. Transcriptome profiling reveals the EanI/R quorum sensing regulon in
Pantoea ananatis LMG 2665T. Genes 9(3). pii: E148.
Serrano, F. B. 1928. Bacterial fruitlet brown-rot of pineapple in the Philippines. Philipp. J. Sci. 36:271-305.
Shyntum, D. Y., Venter, S. N., Moleleki, L. N., Toth, I. and Coutinho, T. A. 2014. Comparative genomics of type VI secretion systems in strains of Pantoea ananatisfrom different environments. BMC Genomics 15:163-178.
Tambong, J. T., Xu, R. L., Kaneza, C. A., Nshogozabahizi, J. C., 2014. An In-depth Analysis of a Multi locus Phylogeny IdentifiesleuS as a Reliable Phylogenetic Marker for the Genus
Pantoea. Evol. Bioinform. 10:115-25.
Tambong, J. T. 2015. Specific identification and detection of
Pantoea stewartii subsp. stewartii using a membrane-based multi-gene oligonucleotide array. Can. J. Plant Pathol.37:414-26.
USDA, 2016. Vegetables 2015 Summary. In: United States Department of Agriculture NASS, ed.
Walcott, R. R., Gitaitis, R. D., Castro, A. C., Sanders, F. H. Jr., and Diaz-Perez, J. C. 2002. Natural infestation of onion seed by
Pantoea ananatis, causal agent of center rot. Plant Dis. 86:106-111.
Wiener, P., Muller-Graf, C., and Barcus, V. 1999. Bacterial evolution in modern times: Trends and implications for research. Integr. Biol. 1:149-160.
Weller‐Stuart, T., De Maayer, P. and Coutinho, T. 2017.
Pantoea ananatis: genomic insights into a versatile pathogen. Mol. Plant Pathol. 18:1191-1198.
Wells, M. L., Gitaitis, R. D., and Sanders, F. H. 2002. Association of tobacco thrips,
Frankliniella fusca (Thysanoptera: Thripidae) with two species of bacteria of the genus Pantoea. Ann. Entomol. Soc. Am. 95:719.
Wells, J. M., Sheng, W. S., Ceponis, M. J., Chen, T. A. 1987. Isolation and characterization of strains of
Erwinia ananas from honeydew melons. Phytopathology 77:511-514.