Lucy R. Stewart
Research Molecular Biologist, Corn and Soybean Research Unit, USDA-ARS
Adjunct Assistant Professor, Department of Plant Pathology, The Ohio State University
1680 Madison Ave. Wooster OH 44691
Contact information: lucy.stewart@ars.usda.gov
Stewart, L. R. 2011. Waikaviruses: Studied but not understood. APS Features. doi:10.1094/APSFeature-2011-11.
What are Waikaviruses?
Maize chlorotic dwarf virus (MCDV) and Rice tungro spherical virus (RTSV) cause important diseases of corn (Zea mays L.) and rice (Oryza sativa L.), respectively. In the mid-1970s, MCDV was widely distributed from the Gulf of Mexico up to southern parts of the Corn Belt states between the southeastern coast and Texas (29). RTSV is one of two viruses that cause rice tungro disease in coinfected plants. Rice tungro disease is the most important virus disease of rice and a major problem in southern and southeast Asia (5). There are only a few known members of the genus Waikavirus, including MCDV and RTSV. Despite being few in number, the waikaviruses have unique properties and biology, as well as major agricultural impact. Information about their fundamental molecular biology is limited because of the difficulties of working with phloem-limited viruses and their insect vectors. With the advantages of knowledge gained since the 1960s about waikaviruses and the diseases they cause, and continued improvements in molecular virology, it is an opportune time to revisit this unique group of viruses.
Rice Tungro
Rice tungro disease (RTD) is the most important viral disease of rice, prevalent throughout southern and southeast Asia (5). The disease has likely caused problems in these areas since at least the 1800s, where it was recognized under various local names (5). Despite losses, the etiology of rice tungro disease was not known for many years. In the 1960s and 1970s RTD incidence increased, corresponding to the time frame of intensified rice cultivation in Asia (5). At this time, several research groups noted leafhopper transmission of RTD, as well as the presence of virus particles in diseased plants (24,25,39,45,62,74,87). In 1978, RTD was associated with two distinct virus particles: a spherical virus and a bacilliform virus, both of which were transmitted by the rice green leafhopper (Nephotettix virescens [Distant]) (34). RTSV has spherical virions (Fig. 1A) and is required for leafhopper transmission of the second virus, Rice tungro bacilliform virus (RTBV), which, in turn, is responsible for most symptoms of the disease (32,35,40). RTBV is a DNA pararetrovirus in the family Caulimoviridae (66). RTBV causes stunting and yellow-orange discoloration on leaves of infected rice plants, symptoms that are enhanced by co-infection with RTSV (Fig 1B). No vector is known to transmit RTBV alone. Without RTBV, most isolates of RTSV cause very mild or no apparent symptoms in most rice genotypes, with the exception of a severe Japanese RTSV formerly termed “rice waika virus” (73). The genus Waikavirus is named for rice waika disease, the name ‘waika’ being a derivative of a Japanese term for ‘stunting’ (51,63).
Fig. 1. Rice tungro spherical virus (RTSV) and Maize chlorotic dwarf virus (MCDV). (A) Transmission electron micrograph of RTSV virions. Bar = 100 nm. (Photograph courtesy of Dr. Filomena Sta Cruz, University of the Philippines-Los Banos). (B) Rice plants infected with RTSV and RTBV (left), RTBV alone (center left), RTSV alone (center right), and healthy (right). (Photograph courtesy of Dr. Filomena Sta Cruz, University of the Philippines-Los Banos). (C) Vein chlorosis in corn infected with MCDV-S. (D) The primary vector of MCDV in the eastern United States, the black-faced leafhopperGraminella nigrifrons. (Photograph courtesy of Jane Todd, USDA-ARS). (E) MCDV-S-infected stunted plants in a pot with a single uninfected plant. |
Control of RTD continues to be challenging. Control of inoculum sources by rogueing infected plants, introducing fallow periods with no adjacent rice to reduce leafhopper populations, and control of leafhoppers by insecticide treatment can all be effective in reducing RTD, but are often impractical (5). Effective screening of rice germplasm for RTD resistance using leafhopper transmission is hampered by disease escapes associated with incomplete transmission. Such screens also do not differentiate between leafhopper and virus resistance, and consequently, released RTD resistant germplasm usually has leafhopper resistance rather than virus resistance (5). While leafhopper resistance is very effective for controlling disease in the short term, it has not been stable because leafhopper populations often rapidly overcome resistance (4,5,15). So far, germplasm resistant to RTSV but not RTBV has been identified (5,42), even though RTBV resistance can be screened separately using agroinoculation (infection by infiltration with Agrobacterium tumefaciens containing plasmid engineered to express infectious virus in plants) instead of insect inoculation (17,76). Transgenic approaches to obtain pathogen-derived resistance (16) have been attempted with some success, but transgenic germplasm has not yet been released for rice production. Sporadic outbreaks of RTD associated with high vector populations still remain problematic (5).
Maize Chlorotic Dwarf
In the 1960s, several new corn diseases made destructive appearances in the United States. Among these was a disease caused by a “stunting agent” and transmitted by leafhoppers, predominantly Graminella nigrifons (Forbes) (60,68) (Fig. 1C-E). By the mid-1970s, this disease was widely distributed from the Atlantic coast westward to Texas and from the Gulf of Mexico to the southern parts of Ohio and the Corn Belt states (29). However, the etiology of the disease was not immediately determined. In 1972, a spherical virus associated with leafhopper-transmitted corn stunting was identified (64). In 1973, the virus was named Maize chlorotic dwarf virus (59,60). The MCDV virus particles were isolated and characterized in 1976, and were shown to have greater buoyant density than any other known virus particles except for RTSV (27).
Johnsongrass (Sorghum halepense Pers.) is a major overwintering host of MCDV that provides inoculum for annual infection of corn seedlings. Therefore, managing Johnsongrass is a major factor for MCDV control (47). In addition to corn and Johnsongrass, MCDV also infects Sudan grass (Sorghum bicolor L. Moench), proso millet (Panicum miliaceum L.), pearl millet (Pennisetum glaucum L.), sorghum (Sorghum vulgare L. Moench), and wheat (Triticum aestivum L.), although it is asymptomatic in wheat and sorghum (26,58). Insecticide treatments have also been tested and recommended for MCDV control, but are usually too costly to be practical (26).
Initial efforts to identify corn germplasm resistant to MCDV provided contradictory results (30,43), possibly because of mixed infections with other viruses and disease escapes (56,69). In addition, introgression of multigenic MCDV resistance from an immune and corn-interfertile teosinte (Zea diploperrenis L.) was attempted (22,26) with some success. Quantitative resistance in germplasm from the Virgin Islands was later identified using a severe MCDV isolate transmitted by leafhoppers under controlled conditions, and scoring for disease severity rather than incidence (41,48,49,65). Whether currently available commercial hybrids utilize published resistance sources is not clear. No data on the current distribution and yield losses caused by MCDV are publicly available, and the risk of future losses from this virus can only be speculated.
Helping Transmission
A very intriguing facet of the biology of waikaviruses is their transmission by insect vectors. Leafhopper transmission of RTSV and MCDV, and aphid transmission of the only other known waikavirus, Anthriscus yellows virus (AYV), are designated as “semi-persistent.” Waikavirus virions are “semi-persistent” in insect vectors because they can be transmitted immediately after acquisition for up to 2-3 days (19,35,60). In contrast, “non-persistent” viruses are retained and transmitted at most for several hours after vector acquisition, and “persistent” viruses are retained for periods ranging from days to the life of the insect vector (61). Persistent viruses are also not transmitted by their vector until a post-acquisition latent period has elapsed (61). Two research groups initially described waikavirus transmission as “transitory,” but the term “semi-persistent” was borrowed from aphid transmission literature and is now widely used (12,26,46,78). Although relatively uncommon among plant viruses, semi-persistent transmission is also described for caulimoviruses and closteroviruses (61). Waikavirus virions are reported to accumulate in leafhopper (MCDV) or aphid (AYV) foreguts (3,11,54) (Fig. 2) rather than on insect stylets as is the case for non-persistently transmitted plant viruses, or throughout the vector or in other specific organs as is the case for persistently transmitted viruses. In contrast to aphid-transmitted plant viruses which may be transmitted persistently, semi-persistently, or non-persistently, all known leafhopper-transmitted plant viruses except for RTSV and MCDV associate with their vectors in a persistent manner (57).
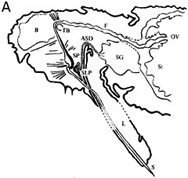 |
|
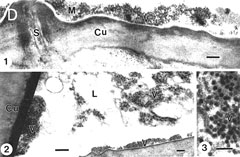 |
|
|
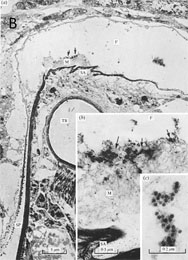 |
|
Fig. 2. Foregut localization of Anthriscus yellows virus (AYV) and Maize chlorotic dwarf virus (MCDV) virions in their insect vectors. (A) “Drawing of a dorso-ventral longitudinal section through the head and thorax of Cavariella aegopodii showing the arrangement of the parts of the anterior alimentary tract, SP = sucking pump; F = foregut; TB = tentorial bar; OV = oesophageal valve; St = stomach; L = labium; SLP = salivary pump; ASD = afferent salivary duct; SG = salivary glands; B = brain; S = stylets.” (B) “(a) Dorso-ventral longitudinal section through the anterior alimentary tract of Cavariella aegopodii which had fed for 24 h on chervil containing AYV and then for 2 h through a membrane on a purified preparation of PYFV in 10% sucrose. The section shows the junction of the sucking pump (SP) with the foregut (F) in the region of the tentorial bar (TB). Virus-like particles (arrows) are embedded in a matrix of M-material (M) overlying the spiked area (SA) of the ventral intima of the foregut. Insets (b) and (c) are progressively greater enlargements of the matrix area. In inset (c) the densely staining material surrounding the virus-like particles is clearly visible.” (A) and (B). Figures and legends reprinted from Journal of General Virology 31, Murant, Roberts, and Elganar, Association of virus-like particles with foregut of aphid Cavariella aegopodii transmiting semi-persistent viruses Anthriscus yellows and Parsnip yellow fleck pp. 47-57, Copyright (1976), with permission of the Society for General Microbiology. (C) “(a) Sagittal view of the head of the leafhopper Graminella nigrifrons showing anterior alimentary canal, salivary system, and surrounding structures. (b) Details of the precibarium and salivary syringe areas. B = brain; cb = cibarium; cdm = cibarial dilator muscle; d = dibarial diaphragm; ds = distal sensilla; e = esophagus; ev = esophageal valve; fc = food canal; Lm = labium; Lr = labrum; MG = midgut; p = precibarium; Ph = pharynx; pr = piston retractor muscle; ps = proximal sensilla; pv = precibarial valve; pvm = precibarial valve muscle; S = stylets; sc = salivary canal; sd = salivary duct; seg = subesophageal ganglion; SG = salivary gland; ss = salivary syringe; tb = tentorial bar (E.D. Ammar, unpublished).” Figure and legend reprinted from Annual Review of Entomology 34, Nault and Ammar, Leafhopper and planthopper transmission of plant viruses pp. 277-285, Copyright (1989), with permission of Annual Reviews, Inc. (D) “Aggregates of viruslike particles (VLP = V) embedded in semiopaque matrix material (M), attached to the cuticular intima (Cu) of parts of the foregut in Graminella nigrifrons (Figs 1-3) . . . Previously exposed to plants infected with maize chlorotic dwarf virus, then fed on healthy maize for 4-5 hr. 1, VLP in the precibarium, above the precibarial valve. S = sensillum. Bar = 200 nm. 2 (and inset), VLP in the cibarium, either attached to the intima (Cu) or floating in the lumen (L). Bars = 200 nm. 3, Highly magnified, matrix-embedded, VLP (V). Bar = 90 nm.” Figure and legend reprinted from Phytopathology 81, Ammar and Nault, Maize chlorotic dwarf virus-like particles associated with the foregut in vector and nonvector leafhopper species pp. 444-448, Copyright (1991), with permission from the American Phytopathological Society. |
Insect transmission of each known waikavirus involves some type of “helper” phenomenon. RTSV is required to “help” transmission of RTBV by an unknown mechanism. RTBV is not transmitted by leafhoppers unless they simultaneously or previously acquired RTSV. This occurs even though RTBV is retained and transmissible in leafhoppers for 1-3 days longer than RTSV after leafhopper acquisition from co-infected plants (31). Membrane feeding of viruliferous leafhoppers to acquire antisera against RTBV or RTSV blocked transmission of the virus targeted by the antisera but not transmission of the second virus (33). These results suggest that a component that is not part of the RTSV virion is required for RTBV transmission.
Evidence for a non-virion associated transmission helper component for MCDV has also been reported. Purified MCDV virions alone are not experimentally transmissible by leafhoppers. However, leafhoppers first fed on plants infected with a mild isolate of MCDV and then on purified virions of a severe MCDV isolate could transmit the severe MCDV isolate (14,37). In contrast, leafhoppers pre-fed on healthy plants or plants infected with the potyvirus Maize dwarf mosaic virus (MDMV) were not able to transmit MCDV virions (14,37). Observation of an electron-dense “matrix” in which MCDV-like virions were embedded in the foreguts of viruliferous G. nigrifrons leafhoppers (Fig. 2D) led one group to suggest that this matrix of unknown composition might comprise the putative virus helper component, allowing the virus to be retained and transmitted by vectors (11). However, subsequently published data showing a similar “matrix” containing virus-like particles in another, nonvector cicadellid leafhopper, Dalbulus maidis (DeLong & Wolcott), suggested that the situation is more complex (3). While the matrix may be a determinant of virus transmission, other factors must also be important in determining leafhopper transmission capability (3). In experiments testing for the presence of MCDV-encoded proteins in leafhoppers, antisera against the 78 kDa MCDV N-terminal polyprotein (Fig. 3) detected a 25 kDa protein (P25) in viruliferous but not naïve leafhoppers (never exposed to virus) (10). Since other non-capsid proteins were not detected in viruliferous leafhoppers using antisera to two other MCDV polyprotein regions, R37 and R69 (Fig. 3), the P25 protein appeared to be a good candidate for the putative “helper component” protein (10).
Fig. 3. RTSV-A and MCDV-S genome organization. Open reading frames are shown to scale in each virus, based on sequences NC_001632 (NCBI; RTSV-A) and AY362551 (NCBI; MCDV-S), respectively. VPg, virus protein genome-linked. Experimentally validated polyprotein cleavage sites are shown as solid lines. Predicted cleavage sites are shown as dashed lines. Green bars indicate transmembrane helices predicted by InterProScan (ORFX; ebi.ac.uk) or SMART (smart.embl-heidelberg.de); red bar indicates predicted coiled-coil, and purple indicates predicted helicase domain. Viral 3C-protease region is indicated in yellow. MCDV polyprotein regions of unknown function R78, R37, and R69 are indicated, with proteins detected using antisera against these polyproteins listed below. |
A “helper” phenomenon has also been described for AYV, which is transmitted by the carrot-willow aphid Cavariella aegopodii (Scop.). AYV “helps” the transmission of the sequivirus Parsnip yellow fleck virus (PYFV); PYFV is only transmitted by aphids simultaneously or previously exposed to AYV. Crude AYV virus preparations or sap from infected plants could not be transmitted by aphids after membrane-feeding, suggesting that some non-capsid component that is lost, diluted, or disrupted in these preparations might be necessary for transmission of AYV itself also (20). Experiments in which UV-inactivated and then infectious PYFV virions were sequentially fed to aphids carrying AYV and moved to healthy plants to assess virus transmission revealed that the two types of AYV virions competed for binding sites in insect vectors (20). It is not clear from these data whether PYFV binds to AYV virions or a non-virion “helper component,” however.
The available results on transmission characteristics of the waikaviruses raise several questions yet to be answered. Among them are: What is the “helping” mechanism involved in waikavirus-associated transmission? Is a homologous virus-encoded component involved in each case? And can this component be blocked to prevent virus transmission?
Cytopathology
Each of the three known waikaviruses is reported to be phloem-limited (2,53,77), although MCDV virions are occasionally present in bundle sheath and mesophyll cells (8). Several cytopathologies are associated with waikavirus infection. Cytopathologies induced by mild (M1) or more severe (T) isolates of MCDV in corn and Johnsongrass include dense granular inclusions (DGIs; Fig. 4) and fibrous inclusions (FIs) in vascular parenchyma and phloem cells, and these inclusions are infrequently found in bundle sheath and mesophyll cells (1). DGIs are associated with virus particles, with more abundant particles in cells infected with the severe compared to the mild strain (1). Inclusion bodies similar to the DGIs were also reported in host plant cells infected with AYV (53) and in plants infected with RTSV (21), and were associated with virus particles and occasionally with lipid droplets. In chervil (Anthriscus cerefolium L. Hoffm.) infected with AYV, cell wall protrusions were associated with virus particles but were not associated with plasmodesmata (53). AYV appeared confined to leaf midveins and central petiole vascular bundles in this host, and was not found in other vasculature (53). Crystalline arrays of RTSV particles were also observed in rice, which, although not recognized at the time, was co-infected with RTBV (21) (Fig. 4A).
Fig. 4. Cytopathologies induced by Rice tungro spherical virus and Maize chlorotic dwarf virus. (A) Rice tungro disease-infected rice leaf at one month post-infection. Upper panel shows phloem parenchyma cell with RTSV particles in the cytoplasm (arrows) and in a large viroplasm (VP) mass. M, mitochondria; L, lipid droplet. Inset: crystalline aggregate of RTSV particles in the vacuole of a phloem parenchyma cell. Lower panel shows companion cell containing many short, coreless tubules (RTBV virions) in different planes of section in the cytoplasm (arrows). RTSV particles are also visible. VP, viroplasms. Inset: Enlargement of RTBV virions. Figure reprinted from Virology 71, Favali, Pellegrini, and Bassi, Ultrastructural alterations induced by rice tungro virus in rice leaves pp. 718-724, Copyright (1975), with permission from Elsevier. Note that the legend is different from original since RTBV bacilliform virions were not recognized at time of original publication. Contrast adjusted. (B) Electron micrograph showing dense granular inclusion (D) in a thin section of a maize leaf infected with the MCDV-T. No virions are apparent in the inclusion. m, mitochondrion. Scale bar = 150 nm. Figure reprinted from Canadin Journal of Botany 71, Ammar, Gingery, and Nault, Cytopathology and ultrastructure of mild and severe strains of Maize chlorotic dwarf virus in maize and johnsongrass pp. 718-724, Copyright (1993), with permission from National Research Council, Canada. |
Extensive starch accumulation was observed in mesophyll chloroplasts of RTSV and RTBV co-infected tissue, which suggested disrupted sugar transport in the infected plants (21). This coincides with some of the physiological effects reported in early literature for RTSV, including decreased soluble sugars and increased starch content in infected plants, as well as decreased free amino acid content (75). Vesiculation, common in cells where RNA virus replication takes place, was also noted for MCDV-infected phloem cells (1).
Symptoms associated with waikavirus infection vary from undetectable (for most RTSV isolates) to strong tertiary vein chlorosis and stunting (MCDV-S) (9,26,36). In contrast to the related but not phloem-limited sequivirus PYFV, waikaviruses do not induce plasmodesmata-traversing, virion-containing tubules in infected cells (55).
Molecular Biology of Waikaviruses
Waikaviruses have monopartite (+) ssRNA genomes encapsidated in icosahedral particles consisting of three different capsid proteins (CP1, CP2, and CP3) (27,28) (Fig. 3). The numerical designation of each capsid protein is not the same as for RTSV and MCDV; RTSV capsids were named in coding order while MCDV capsids were named in order of decreasing molecular weight (50,72). Waikavirus virions have high buoyant densities (1.507 g/mL in CsCl for MCDV) and rapid sedimentation rates (154 S for MCDV and 175 S for RTSV) relative to other plant viruses (24,27).
Genomic RNAs are ca. 12-12.5 kb, polyadenylated, and predicted to have an attached 5’ VPg (viral protein, genome-linked). Waikavirus genomes encode a large polyprotein of approximately 390 kDa, which is post-translationally cleaved into mature proteins (67,72). Sequences indicative of a helicase, a 3C-like viral cysteine/serine protease, and an RNA-dependent RNA polymerase are predicted in waikavirus genomes (9,67,72). Additional small open reading frames (ORFs) are also predicted, but the number and location of these are variable between MCDV and RTSV, and even variable among isolates of each virus (9,67,72,81). A small overlapping ORF (ORFX) is predicted in the waikaviruses that is conserved across MCDV and RTSV sequences (23). In addition, waikaviruses have a long 5’ untranslated region (UTR), which contains multiple AUG codons upstream of the predicted start site (9,18). These 5’ UTRs have IRES (internal ribosome entry site) sequence signatures (9,72) similar to plant picornaviruses, which may allow translation of the viral genome by recruiting host ribosomes in the absence of a 5’ 7-methylguanosine cap. Experimental evidence for waikavirus translation mechanisms, however, is lacking.
Sequences
The genomic features of waikaviruses align them with eukaryotic virus order Picornavirales. These features include a positive sense RNA genome with a putative 5’ VPg and 3’ polyadenylation, proteolytically processed polyprotein expression strategy, icosahedral capsid structure, a superfamily III helicase, a cysteine protease and an RNA-dependent RNA polymerase (44). Originally, waikaviruses were classified in the family Sequiviridae, along with the genus Sequivirus, in which PYFV is the type and only member. More recently, they have been reclassified to combine the former families Sequiviridae and Comoviridae with the formerly unclassified genera Cheravirus and Sadwavirus into the new family Secoviridae (70). The family Secoviridae also encompasses a new genus of recently-discovered emerging viruses, Torradovirus (70,82,83,84). The family Secoviridae is comprised of eight genera: Comovirus, Fabavirus, Nepovirus, Cheravirus, Sadwavirus, Torradovirus, Sequivirus, and Waikavirus (70).
Four complete sequences are now available for RTSV (38,72,85), and four for MCDV (9,52,67) (R. Gingery, unpublished; Table 1). Herein, these sequences are termed “isolates,” a subset of which have been demarcated as separate “strains” in the literature based on symptom severity or sequence divergence. Complete RTSV sequences include the type strain (RTSV-A), a West Bengal isolate, an Orissa isolate, and a virulent Vt6 strain. Nucleotide sequence identities are 96% for the RTSV West Bengal and Orissa isolates, and 89 to 90% identity between the RTSV-A and each of the other RTSV full-length sequences (Table 1). In addition, three 3’ end sequences and multiple 5’ partial sequences in the CP region are also published for RTSV (81,88,89). Based on five partial CP1 sequences, two distinct clades of RTSV were described, one subgroup of viruses from the Indian subcontinent and one from Malaysia and the Philippines, with an intermediate Thai isolate (88,89). Sequence analysis of more RTSV isolates, mostly collected in the Philippines and India, indicated that multiple clades of RTSV exist, and cluster by location (6) (Fig. 5).
Fig. 5. Phylogenetic analysis of Rice tungro spherical partial CP1 sequences. Tree based on partial CP1 nucleic acid sequences corresponding to nt 2477-3070 of RTSV-A (GenBank accession number M95497.1). Corresponding sequences from Tomato torradovirus (ToTV), family Secoviridae genus Torradovirus and MCDV-S coat proteins were also included. Tree constructed by bootstrap analysis (1000 replicates) using neighbor-joining method in MacVector (v. 7.2.3; Cary, NC). Sequences are named according to virus collection location where known, sequence name, and GenBank accession number. Philippines: Pe, Nueva Ecija; Pc, North Cotabato; Pi, IRRI. Indonesia: Is, Subang; Ib, Bali island. India: Na, Andra Pradesh; Nt, Tamil Nadu-Coimbatore; Nw, West Bengal; No, Orissa. Malaysia: Ma. Isolates for which complete genome sequences are available are indicated with arrows. |
Sequence surveys or phylogenetic studies have not been performed for MCDV. Sequenced MCDV genomes include the type (MCDV-T) and mild (MCDV-M1) strains, and severe (MCDV-S) and Tennessee (MCDV-Tn) isolates (9,52,67) (R. Gingery, unpublished). The nucleotide sequences for MCDV-S and MCDV-T are 99% identical, while MCDV-Tn and MCDV-M1 sequences are only 65% identical to each other, and only 56% and 57% identical, respectively, to MCDV-T. In summary, the four RTSV complete nucleotide sequences are quite similar to one another, in contrast to the MCDV sequences which have much greater variability (Table 1).
Polyprotein Cleavage
Waikaviruses have a 3C-like protease at the C-terminus of the polyprotein, just upstream of the polymerase motifs. This protease has conserved catalytic amino acids similar to those of the potyvirus 3C-like proteases (79). No other trans-acting proteases are predicted in waikavirus genomes, but a predicted autocatalytic site in the N-terminal region of MCDV is supported by protein detection data using polyprotein antiserum (10). A conserved cleavage recognition site, if any, for the 3-C protease remains to be determined, although glutamine residues (Q) are often favored in the -1 position relative to the scission site for cysteine/serine viral proteases (7), and -1 Q residues are present in the experimentally determined waikavirus scission sites (67,71,72,79,80).
The N-terminal amino acids for RTSV and MCDV coat proteins have been sequenced, each preceded by a -1 Q residue (67,80). However, in vitro experiments attempting to show cleavage of the three RTSV CPs using active RTSV protease were not successful (79). N- and C-terminal sites for autocatalytic release of the protease have been determined for RTSV using an in vitro system, and an internal cleavage site of the RTSV protease has also been demonstrated (67). However, none of the identified RTSV protease self-cleavage sites are conserved in MCDV sequences. Other protease cleavage sites within the polyprotein have not been experimentally determined.
Small ORFs
Two small 3’ ORFs are predicted in the RTSV-A type isolates, and corresponding 3’ subgenomic RNAs from which they might be expressed were observed in northern blots (67). However, partial genome sequence for two other RTSV isolates had stop codons within these RTSV-A predicted ORFs, and attempts to detect the predicted protein products were not successful (81). MCDV-S has predicted 5’ and 3’ small ORFs outside of the polyprotein sequence, but these are also variable among sequences (9). Whether any of these small ORFs encode expressed proteins is unknown. Another small “ORFX ”overlapping the 5’ end of the polyprotein ORF is predicted for all waikavirus sequences (23) (Fig. 3), but expression of an encoded protein has not been confirmed. In contrast to the potyvirus PIPO (pretty interesting Potyviridae ORF) (13,86), the waikavirus ORFX has no upstream sequence predicted to facilitate frameshifting, and begins with an in-frame AUG codon (23).
Functions of Virus Proteins
Sequences of waikavirus capsid proteins and predicted helicase, protease, and RNA-dependent RNA polymerase have been identified (9,38,67,72). Functions of proteins encoded by the remainder of the polyprotein remain to be experimentally determined. Identification of proteins using antisera against each of three polyprotein regions of unknown function provided some clues as to their expression in plant tissue and cleavage to mature proteins (10). For example, antisera against the 78 kDa N-terminal polyprotein of MCDV-S detected proteins of 50, 35, and 25 kDa in infected plant tissue, and the 25 kDa protein was also detected in viruliferous leafhoppers using this antiserum. This suggested possible cleavage locations for the 78 kDa protein and potential transmission helper component function for the P25 protein product (10) (Fig. 3). Until sites of proteolysis are identified and expression of predicted small ORFs is tested, the full suite of mature waikavirus proteins remains unknown. Currently, no full-length infectious clones have been produced, making genetic analysis of virus gene function prohibitive. Future information on which putative viral proteins are expressed and the functions of individual proteins, as well as elucidation of the unusual transmission features of waikaviruses will be very useful in understanding the basic biology of these viruses.
Future Directions for Waikavirus Research
Great strides in characterization of waikaviruses have been made since researchers in the 1960s and 1970s identified them as causal agents of important rice and corn diseases. Making these advances for the waikaviruses was especially challenging because of their presence in mixed infections, as well as their insect transmission and phloem-limited nature, because phloem-limited insect-transmitted viruses are usually present at lower titer and are not readily mechanically transmissible to test plants. The next steps for waikavirus research include continued development of virus resistant corn and rice germplasm, improving our understanding of transmission mechanisms, and continuing basic virological research to elucidate waikavirus diversity and distribution, gene expression and protein function for these important viruses.
Acknowledgments
Sincere thanks to Jane Todd and Drs. Margaret. G. Redinbaugh, Donald. T. Gordon, Gary D. Franc, Filomena C. Sta Cruz, and Roger Hull for critical reading of the manuscript, and to Drs. Raymond Louie and Lowell R. Nault for helpful discussions.
Literature Cited
1. Ammar, E. D., Gingery, R. E., and Nault, L. R. 1993. Cytopathology and ultrastructure of mild and severe strains of Maize chlorotic dwarf virus in maize and Johnsongrass. Can. J. Bot. 71:718-724.
2. Ammar, E. D., Gordon, D. T., and Nault, L. R. 1987. Ultrastructure of Maize chlorotic dwarf virus-infected maize and viruliferous leafhopper vectors. Phytopathology 77:1743-1743.
3. Ammar, E. D., and Nault, L. R. 1991. Maize chlorotic dwarf virus-like particles associated with the foregut in vector and nonvector leafhopper species. Phytopathology 81:444-448.
4. Angeles, E. R., and Khush, G. S. 2000. Genetic analysis of resistance to green leafhopper, Nephotettix virescens (Distant), in three varieties of rice. Plant Breed. 119:446-448.
5. Azzam, O., and Chancellor, T. C. B. 2002. The biology, epidemiology, and management of rice tungro disease in Asia. Plant Dis. 86:88-100.
6. Azzam, O., Yambao, M. L. M., Muhsin, M., McNally, K. L., and Umadhay, K. M. L. 2000. Genetic diversity of Rice tungro spherical virus in tungro-endemic provinces of the Philippines and Indonesia. Arch. Virol. 145:1183-1197.
7. Bazan, J. F., and Fletterick, R. J. 1988. Viral cysteine proteases are homologous to the trypsin-like family of serine proteases: Structural and functional implications. Proc. Natl. Acad. Sci. U. S. A. 85:7872-7876.
8. Bradfute, O. E., Gingery, R. E., Gordon, D. T., and Nault, L. R. 1972. Tissue ultrastructure, sedimentation and leafhopper transmission of a virus associated with a maize stunting disease. J. Cell Biol. 55:25a.
9. Chaouch, R., Redinbaugh, M. G., Marrakchi, M., and Hogenhout, S. A. 2004. Genomics of the severe isolate of Maize chlorotic dwarf virus. Plant Prot. Sci. 40:113-119.
10. Chaouch-Hamada, R., Redinbaugh, M. G., Gingery, R. E., Willie, K., and Hogenhout, S. A. 2004. Accumulation of maize chlorotic dwarf virus proteins in its plant host and leafhopper vector. Virology 325:379-388.
11. Childress, S. A., and Harris, K. F. 1989. Localization of virus-like particles in the foreguts of viruliferous graminella nigrifrons leafhoppers carrying the semi-persistent maize chlorotic dwarf virus. J. Gen. Virol. 70:247-251.
12. Choudhury, M. M., and Rosenkranz, E. 1983. Vector relationship of Graminella nigrifrons to Maize chlorotic dwarf virus. Phytopathology 73:685-690.
13. Chung, B. Y., Miller, W. A., Atkins, J. F., and Firth, A. E. 2008. An overlapping essential gene in the Potyviridae. Proc. Natl. Acad. Sci. U. S. A. 105:5897-5902.
14. Creamer, R., Nault, L. R., and Gingery, R. E. 1993. Biological factors affecting leafhopper transmission of purified maize chlorotic dwarf machlovirus. Entomol. Exp. Appl. 67:65-71.
15. Dahal, G., Hibino, H. Cabunagan, R. C., Tiongco, E. R., Flores, Z. M., and Aguiero, V. M. 1990. Changes in cultivar reactions to tungro due to changes in virulence of the leafhopper vector. Phytopathology 80:659-665.
16. Dai, S. H., and Beachy, R. N. 2009. Genetic engineering of rice to resist rice tungro disease. In Vitro Cell. Dev. Biol.: Plant 45:517-524.
17. Dasgupta, I., Hull, R., Eastop, S., Poggipollini, C., Blakebrough, M., Boulton, M. I., and Davies, J. W. 1991. Rice tungro bacilliform virus-dna independently infects rice after agrobacterium-mediated transfer. J. Gen. Virol. 72:1215-1221.
18. Druka, A., Zhang, S., Yan, Y., Burns, T. M., and Hull, R. 1995. Investigation of the leader sequence and upstream genes of Rice tungro spherical virus. Pages 857-861 in: Rice Genetics III: Proceedings of the Third International Rice Genetics Symposium, Manila, Philippines, 16-20 October 1995. G. S. Khush, ed. Intl. Rice Res. Inst., Manila, Philippines.
19. Elnagar, S., and Murant, A. F. 1976. Relations of semi-persistent viruses, parsnip yellow fleck and anthriscus yellows, with their vector, Cavariella aegopodii. Ann. Appl. Biol. 84:153-167.
20. Elnagar, S., and Murant, A. F. 1976. Role of helper virus, anthriscus yellows, in transmission of parsnip yellow fleck virus by aphid Cavariella aegopodii. Ann. Appl. Biol. 84:169-181.
21. Favali, M. A., Pellegrini, S., and Bassi, M. 1975. Ultrastructural alterations induced by rice tungro virus in rice leaves. Virology 66:502-507.
22. Findley, W. R., Nault, L. R., Styer, W. E., and Gordon, D. T. 1983. Zea diploperennis as a source of Maize chlorotic dwarf virus resistance. Proceedings International Maize Virus Disease Colloquium and Workshop, The Ohio State University, Ohio Agricultural Research and Development Center, Wooster OH.
23. Firth, A. E., and Atkins, J. F. 2008. Bioinformatic analysis suggests that a conserved ORF in the waikaviruses encodes an overlapping gene. Arch. Virol. 153:1379-1383.
24. Galvez, G. E. 1968. Purification and characterization of rice tungro virus by analytical density-gradient centrifugation. Virology 35:418-426.
25. Galvez, G. E. 1967. Purification of virus-like particles from rice tungro virus-infected plants. Virology 33:357-359.
26. Gingery, R. E. 1988. Maize chlorotic dwarf and related viruses. Pages 259-272 in: The Plant Viruses, Vol. 3. R. Koenig, ed. Plenum Press, New York, NY.
27. Gingery, R. E. 1976. Properties of Maize chlorotic dwarf virus and its ribonucleic acid. Virology 73:311-318.
28. Gingery, R. E., and Nault, L. R. 1990. Severe maize chlorotic dwarf disease caused by double infection with mild virus strains. Phytopathology 80:687-691.
29. Gordon, D. T., and Nault, L. R. 1977. Involvement of maize chlorotic dwarf virus and other agents in stunting diseases of Zea mays in United States. Phytopathology 67:27-36.
30. Guthrie, W. D., Tseng, C. T., Knoke, J., and Jarvis, J. L. 1982. European corn-borer (Lepidoptera, Pyralidae) and Maize chlorotic dwarf virus resistance-susceptibility in inbred lines of dent maize. Maydica 27:221-233.
31. Hibino, H. 1996. Biology and epidemiology of rice viruses. Annu. Rev. Phytopathol. 34:249-274.
32. Hibino, H. 1983. Transmission of two Rice tungro-associated viruses and Rice waika virus from doubly or singly infected source plants by leafhopper vectors. Plant Dis. 67:774-777.
33. Hibino, H., and Cabauatan, P. Q. 1987. Infectivity neutralization of rice tungro-associated viruses acquired by vector leafhoppers. Phytopathology 77:473-476.
34. Hibino, H., Roechan, M., and Sudarisman, S. 1978. Association of two types of virus particles with Penyakit Habang (tungro disease) of rice in Indonesia. Phytopathology 68:1412-1416.
35. Hibino, H., Saleh, N., and Roechan, M. 1979. Transmission of two kinds of rice tungro-associated viruses by insect vectors. Phytopathology 69:1266-1268.
36. Hull, R. 1996. Molecular biology of rice tungro viruses. Annu. Rev. Phytopathol. 34:275-297.
37. Hunt, R. E., Nault, L. R., and Gingery, R. E. 1988. Evidence for infectivity of Maize chlorotic dwarf virus and for a helper component in its leafhopper transmission. Phytopathology 78:499-504.
38. Isogai, M., Cabauatan, P. Q., Masuta, C., Uyeda, I., and Azzam, O. 2000. Complete nucleotide sequence of the Rice tungro spherical virus genome of the highly virulent strain Vt6. Virus Genes 20:79-85.
39. John, V. T. 1968. Identification and characterization of tungro, a virus disease of rice in India. Plant Dis. Rep. 52:871-875.
40. Jones, M. C., Gough, K., Dasgupta, I., Rao, B. L. S., Cliffe, J., Qu, R., Shen, P., Kaniewska, M., Blakebrough, M., Davies, J. W., Beachy, R. N., and Hull, R. 1991. Rice tungro disease is caused by an RNA and a DNA virus. J. Gen. Virol. 72:757-761.
41. Jones, M. W., Redinbaugh, M. G., Anderson, R. J., and Louie, R. 2004. Identification of quantitative trait loci controlling resistance to Maize chlorotic dwarf virus. Theor. Appl. Genet. 110:48-57.
42. Khush, G. S., Angeles, E., Virk, P. S., and Brar, D. S. 2004. Breeding rice for resistance to tungro virus at IRRI. SABRAO J. Plant Breed. Genet. 36:101-106.
43. Knoke, J. K., Anderson, R. J., Findley, W. R., Louie, R., Abt, J. J., and Gordon, D. T. 1981. The reaction of sweet corn hybrids to Maize chlorotic dwarf virus strains and Maize chlorotic dwarf virus. Ohio Agr. Res. Dev. Center Res. Bull. 3-22.
44. Le Gall, O., Christian, P., Fauquet, C. M., King, A. M. Q., Knowles, N. J., Nakashima, N., Stanway, G., and Gorbalenya, A. E. 2008. Picornavirales, a proposed order of positive-sense single-stranded RNA viruses with a pseudo-T=3 virion architecture. Arch. Virol. 153:715-727.
45. Ling, K. C. 1966. Nonpersistence of tungro virus of rice in its leafhopper vector Nephotettix impicticeps. Phytopathology 56:1252-1256.
46. Ling, K. C., and Tiongco, E. R. 1979. Transmission of rice tungro virus at various temperatures: A transitory virus-vector interaction. Pages 349-366 in: Leafhopper Vectors and Plant Disease. K. Maramorosch and K. F. Harris, eds. Academic Press, New York, NY.
47. Louie, R. 1999. Maize chlorotic dwarf. Pages 51-54 in: Compendium of Corn Diseases, 3rd Edn. D. G. White, ed. The American Phytopathological Society, St. Paul, MN.
48. Louie, R., and Anderson, R. J. 1993. Evaluation of Maize chlorotic dwarf virus resistance in maize with multiple inoculations by Graminella nigrifrons (Homoptera, Cicadellidae). J. Econ. Entomol. 86:1579-1583.
49. Louie, R., Jones, M. W., Anderson, R. J., and Redinbaugh, M. G. 2002. Registration of maize germplasm Oh1VI. Crop Sci. 42:991-991.
50. Maroon, C. M., Gordon, D. T., and Gingery, R. E. 1989. Serological relationships of the capsid proteins of the type isolate of Maize chlorotic dwarf virus MCDV-T. Phytopathology 79:1157.
51. Mayo, M. A., and Martelli, G. P. 1993. Virus taxonomy update. Arch. Virol. 133:491-498.
52. McMullen, M. D., Roth, B. A., and Townsend, R. 1996. Maize chlorotic dwarf virus and resistance thereto. USA patent 5569828. U.S. Patent Office, Washington, DC.
53. Murant, A. F., and Roberts, I. M. 1977. Virus-like particles in phloem tissue of chervil (Anthriscus cerefolium) infected with anthriscus yellows virus. Ann. Appl. Biol. 85:403-406.
54. Murant, A. F., Roberts, I. M., and Elnagar, S. 1976. Association of virus-like particles with foregut of aphid Cavariella aegopodii transmitting semi-persistent viruses anthriscus yellows and parsnip yellow fleck. J. Gen. Virol. 31:47-57.
55. Murant, A. F., Roberts, I. M., and Hutcheson, A. M. 1975. Effects of parsnip yellow fleck virus on plant cells. J. Gen. Virol. 26:277-285.
56. Naidu, B., and Josephson. L. M. 1976. Genetic analysis of resistance to corn virus disease complex. Crop Sci. 16:167-172.
57. Nault, L. R., and Ammar, E. D. 1989. Leafhopper and planthopper transmission of plant viruses. Annu. Rev. Entomol. 34:503-529.
58. Nault, L. R., Gordon, D. T., Robertson, D. C., and Bradfute, O. E. 1976. Host range of Maize chlorotic dwarf virus. Plant Dis. Rep. 60:374-377.
59. Nault, L. R., and Madden, L. V. 1988. Phylogenetic relatedness of Maize chlorotic dwarf virus leafhopper vectors. Phytopathology 78:1683-1687.
60. Nault, L. R., Styer, W. E., Knoke, J. K., and Pitre, H. N. 1973. Semipersistent transmission of leafhopper-borne Maize chlorotic dwarf virus. J. Econ. Entomol. 66:1271-1273.
61. Ng, J. C., and Falk, B. W. 2006. Virus-vector interactions mediating nonpersistent and semipersistent transmission of plant viruses. Annu. Rev. Phytopathol. 44:183-212.
62. Nishi, Y., Kimura, T., and Maejima, I. 1975. Causal agent of “waika” disease of rice plants in Japan. Ann. Phytopathol. Soc. Jpn. 41:223-227.
63. Ou, S. H. 1985. Waika. Pages 43-44 in: Rice Diseases. The Cambrian News, Aberystwyth, Wales, UK.
64. Pirone, T. P., Poneleit, C. G., Freytag, P. H., Bradfute, O. E., and Lung, M. C. Y. 1972. Virus-like particles associated with a leafhopper-transmitted disease of corn in Kentucky. Plant Dis. Rep. 56:652-656.
65. Pratt, R. C., Anderson, R. J., Louie, R., Mcmullen, M. D., and Knoke, J. K. 1994. Maize responses to a severe isolate of Maize chlorotic dwarf virus. Crop Sci. 34:635-641.
66. Qu, R. D., Bhattacharyya, M., Laco, G. S., De Kochko, A., Rao, B. L., Kaniewska, M. B., Elmer, J. S., Rochester, D. E., Smith, C. E., and Beachy, R. N. 1991. Characterization of the genome of rice tungro bacilliform virus: Comparison with Commelina yellow mottle virus and caulimoviruses. Virology 185:354-364.
67. Reddick, B. B., Habera, L. F., and Law, M. D. 1997. Nucleotide sequence and taxonomy of Maize chlorotic dwarf virus within the family Sequiviridae. J. Gen. Virol. 78:1165-1174.
68. Rosenkranz, E. 1969. A new leafhopper-transmissible corn stunt disease agent in ohio. Phytopathology 59:1344-1346.
69. Rosenkranz, E., and Scott, G. E. 1987. Type of gene action in the resistance to Maize chlorotic dwarf virus in corn. Phytopathology 77:1293-1296.
70. Sanfacon, H., Wellink, J., Le Gall, O., Karasev, A., van der Vlugt, R., and Wetzel, T. 2009. Secoviridae: A proposed family of plant viruses within the order Picornavirales that combines the families Sequiviridae and Comoviridae, the unassigned genera Cheravirus and Sadwavirus, and the proposed genus Torradovirus. Arch. Virol. 154:899-907.
71. Sekiguchi, H., Isogai, M., Masuta, C., and Uyeda, I. 2005. 3C-like protease encoded by Rice tungro spherical virus is autocatalytically processed. Arch. Virol. 150:595-601.
72. Shen, P., Kaniewska, M., Smith, C., and Beachy, R. N. 1993. Nucleotide sequence and genomic organization of Rice tungro spherical virus. Virology 193:621-630.
73. Shinkai, A. 1977. Rice waika: New virus-disease, and problems related to its occurrence and control. JARQ (Jpn. Agric. Res. Q.) 11:151-155.
74. Singh, K. G. 1969. Virus vector relationship in penyakit merah of rice. Ann. Phytopathol. Soc. Jpn. 35:322-324.
75. Sridhar, R., Anjaneyulu, A., and Reddy, P. R. 1976. Physiology of rice tungro virus disease: Changes in chlorophyll, carbohydrates, amino acids and phenol contents. J. Phytopathol. (Phytopathol. Z.) 86:136-143.
76. Sta Cruz, F. C., Boulton, M. I., Hull, R., and Azzam, O. 1999. Agroinoculation allows the screening of rice for resistance to rice tungro bacilliform virus. J. Phytopathol. (Phytopathol. Z.) 147:653-659.
77. Sta Cruz, F. C., Koganezawa, H., and Hibino, H. 1993. Comparative cytology of rice tungro viruses in selected rice cultivars. J. Phytopathol. (Phytopathol. Z.) 138:274-282.
78. Sylvester, E. S. 1969. Virus transmission by aphids-viewpoint. Pages 159-173 in: Viruses, Vectors, and Vegetation. K. Maramorosch, ed. Academic Press, New York, NY.
79. Thole, V., and Hull, R. 2002. Characterization of a protein from Rice tungro spherical virus with serine proteinase-like activity. J. Gen. Virol. 83:3179-3186.
80. Thole, V., and Hull, R. 1998. Rice tungro spherical virus polyprotein processing: Identification of a virus-encoded protease and mutational analysis of putative cleavage sites. Virology 247:106-114.
81. Thole, V., and Hull, R. 1996. Rice tungro spherical virus: Nucleotide sequence of the 3' genomic half and studies on the two small 3' open reading frames. Virus Genes 13:239-246.
82. Turina, M., Ricker, M. D., Lenzi, R., Masenga, V., and Ciuffo, M. 2007. A severe disease of tomato in the Culiacan area (Sinaloa, Mexico) is caused by a new picorna-like viral species. Plant Dis. 91:932-941.
83. Verbeek, M., Dullemans, A. M., van den Heuvel, J. F. J. M., Maris, P. C., and van der Vlugt, R. A. A. 2007. Identification and characterisation of tomato torrado virus, a new plant picorna-like virus from tomato. Arch. Virol. 152:881-890.
84. Verbeek, M., Dullemans, A. M., van den Heuvel, J. F. J. M., Maris, P. C., and van der Vlugt, R. A. A. 2008. Tomato marchitez virus, a new plant picorna-like virus from tomato related to tomato torrado virus. Arch. Virol. 153:127-134.
85. Verma, V., and Dasgupta, I. 2007. Sequence analysis of the complete genomes of two Rice tungro spherical virus isolates from India. Arch. Virol. 152:645-648.
86. Wen, R. H., and Hajimorad, M. R. 2010. Mutational analysis of the putative pipo of soybean mosaic virus suggests disruption of PIPO protein impedes movement. Virology 400:1-7.
87. Yokoyama, S., and Sakai, H. 1975. Transmission by green leafhopper, Nephotettix cincticeps Uhler in new dwarf disease of rice plants. Ann. Phytopathol. Soc. Jpn. 41:219-222.
88. Zhang, S., Lee, G., Davies, J. W., and Hull, R. 1997. Variation in coat protein genes among five geographically different isolates of rice tungro spherical virus. Arch. Virol. 142:1873-1879.
89. Zhang, S. L., Davies, J. W., and Hull, R. 1997. Sequences of the three coat protein genes of a Malaysian isolate of Rice tungro spherical virus reveal a close relationship to the Philippine isolate. Virus Genes 15:61-64.