The Mollicutes: Phytoplasmas and Spiroplasmas
History
Plant "yellows" diseases are characterized by chlorotic foliage and general unthriftiness (Figure 1). Other symptoms, such as floral asymmetry and virescence, vascular necrosis, shoot or bud proliferation, stunting and death, may also occur. The causal agents of these diseases were long believed to be viruses because the symptoms were similar to those caused by viruses, no microbe could be isolated consistently, the agents were able to pass through filters that retained bacteria, and no recognizable microbe could be associated with the symptoms by light microscopy. Finally, like some viruses, the agents often were transmissible by grafting and/or by insect vectors. It was not until the 1960s that some yellows diseases were correctly attributed to prokaryotic plant pathogens.
|
Figure 1. |
Much credit for outstanding early work on yellows diseases is due to L.O. Kunkel, a plant pathologist whose careful and dedicated research on aster yellows spanned the mid-1920s to the mid-1950s. Kunkel proved that the agent of aster yellows was infectious and was transmitted in nature by phloem-feeding leafhoppers. Familiar with insect-transmitted viruses, Kunkel was able to determine minimum acquisition, incubation and inoculation access periods for the aster yellows pathogen in its leafhopper vector. In an ingenious set of experiments, he showed that heating inoculative leafhoppers to a sub-lethal temperature destroyed their ability to transmit the pathogen. However, when those non-transmitting insects were then allowed a fresh incubation period the ability to transmit the pathogen was restored. His data proved that the agent propagated in the insect vector. For his many contributions L.O. Kunkel has been called the "Father of Plant and Insect Mycoplasmology."
However, the realization that not all plant yellows agents were viruses had to await the now-legendary experiments of Japanese plant pathologists studying the devastating mulberry dwarf disease. In the late 1960s Doi, hoping to glimpse the elusive "virus" that caused this problem, pored over plant tissue sections visualized with the electron microscope but could find no virus-like particles. Serendipitously, one session at the print developer was interrupted by a veterinary microbiologist developing photographs of the animal pathogen
Mycoplasma gallisepticum. It was Koshimizu who noticed the similarities between his mycoplasma pictures and the odd, mitochondria-sized bodies in Doi's photographs. Properly skeptical, Doi nonetheless took Koshimizu's suggestion to see whether the antibiotic tetracycline would affect disease development. When the antibiotic led to symptom remission, a new field of plant pathology had been born. Although they were initially called pleuropneumonia-like organisms (PPLOs) or mycoplasma-like organisms (MLOs) because of their superficial similarity to that group, these pathogens have since been shown to differ significantly from mycoplasmas and have been designated
phytoplasmas (Sears and Kirkpatrick 1994). Soon after their discovery, many other diseases once assumed to be of viral etiology, but for which no viral agent had been found, were re-examined and in the following years the number of known phytoplasmas and phytoplasma-induced diseases has continued to grow. Although the inability to culture these organisms has limited their characterization, application of recent molecular technology has resulted in a rapid expansion of our knowledge of phytoplasmas and in the establishment of a "candidate" genus,
Candidatus Phytoplasma.
Around 1970, investigation of corn stunt, a significant disease in the tropics and subtropics, was the impetus for the discovery of still another group of related plant pathogens. Davis and Worley at the USDA-ARS in Beltsville, MD were trying to identify the causal agent of corn stunt. When they examined plant filtrates with a light microscope they saw tiny, helical cells that lacked cell walls (Figure 2). They later named these novel microbes
spiroplasmas. Unlike the phytoplasmas, spiroplasmas could be cultivated in artificial medium, making their characterization more convenient than that of phytoplasmas.
|
Figure 2 |
Biology
Phytoplasmas, spiroplasmas, mycoplasmas and their relatives, all prokaryotes lacking cell walls and sharing other unusual cytological and molecular features, together make up the class
Mollicutes ("molli" - soft; "cute" - skin). Although superficially similar to "L-forms" of walled bacteria, which have lost part or all of their cell walls, the mollicutes are taxonomically distinct. Early on, investigators proposed that mollicutes were primitive microbes, possibly the descendents of bacteria that existed before the development of a peptidoglycan cell wall. Later molecular analyses, however, clearly showed that the mollicutes were actually derivatives of Gram positive walled bacterial precursors. The group comprises the smallest and simplest self-replicating cellular organisms, ranging from 0.3-1.2 µm in diameter and having correspondingly small genomes (18-109 daltons).
The mollicutes share several unique features. Because they lack a rigid envelope and are bounded only by a plasmalemma they are pleomorphic, their morphology is influenced by their environment, they are osmotically fragile, and they resist antibiotics, such as penicillin, that target cell wall formation. Mycoplasmas and spiroplasmas, but not phytoplasmas, are cultivable on artificial media, but their recalcitrance to cultivation and the requirement for sterols and other unusual ingredients in the growth medium of many of them has led to their description as "fastidious."
The fact that mollicutes have the smallest known genomes has made this group a target for genomics projects. The record is currently held by a phytopathogenic bermudagrass phytoplasma at 530 kb; in contrast, the genome of
Escherichia coli is about 4600 kb. The
Mycoplasma genitalium genome (580 kb) was the first microbial genome to be completely sequenced. Several other mycoplasma genomes are now available, that of
Spiroplasma kunkelii is nearing completion (http://www.genome.ou.edu/spiro.html), and those of other mycoplasmas, spiroplasmas and phytoplasmas are in progress. Molecular aspects of mollicute biology have been reviewed recently by Razin et al. (1998). The genomes of these prokaryotes have a low G+C content (23-41%). Spiroplasmas and mycoplasmas, but not phytoplasmas, utilize the nucleotide codon UGA to encode the amino acid tryptophan rather than as a translational stop codon as it is in most living things. The genome has sustained a number of deletions during its size reduction, some of which are undoubtedly related to adaptations to a parasitic and pathogenic lifestyle in which certain functions are no longer required. Additional genetic information, however, is present in many mollicutes as extrachromosomal DNA (viruses and plasmids) (Figure 3), sequences of which apparently integrate freely into the genome and cause various types of genomic rearrangements.
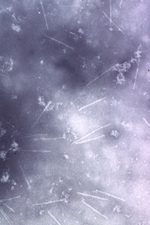 |
Figure 3. |
Spiroplasmas (Family
Spiroplasmataceae) are usually helical in morphology, measuring 0.08 - 0.2 µm wide and 2-4 µm long. Plant pathogenic species may lose helicity when in their insect vector. Motility includes translational movement (including taxes based on gradients of chemicals, temperatures and other environmental factors), rotation about a central axis and a bending/tumbling motion.
Since the discovery of
S. kunkelii in corn plants showing symptoms of "corn stunt" disease (Figure 4) in the 1970s, spiroplasmas have been implicated in other important plant diseases. The causal agent of citrus "stubborn" disease, in which affected trees develop a stunted growth habit, reduced leaf size and asymmetrical and bitter-tasting fruit, was identified as a spiroplasma and designated
S. citri. This same species was later isolated from Illinois horseradish plants with "brittleroot" disease (Figure 5), characterized by phloem necrosis, stunting, and the root fragility from which its name derives. The third known phytopathogenic spiroplasma,
S. phoeniceum, was isolated from periwinkle plants planted, as traps for
S. citri, among the trees in a Syrian citrus grove. The spiroplasma isolated from these plants was distinctly different from
S. citri, but its natural plant host(s) and vector(s) are unknown.
|
|
Figure 4. |
Figure 5. |
Although the first spiroplasmas discovered were plant pathogens, non-phytopathogenic species were quickly identified occupying niches as epiphytes, or causing various diseases in animals, including humans. By far, however, the greatest number reside in insects. The fact that mosquitoes, ticks, honeybees,
Drosophila, beetles, leafhoppers, flies and many other insect species harbor spiroplasmas led Hackett and Clark (1989) to suggest that these organisms may be one of the most prevalent organisms on earth. Because the microbes often cause negative effects on the insect they are potentially useful for biocontrol.
Host-Pathogen Interactions
Spiroplasmas and phytoplasmas cause over 600 diseases in several hundred plant species (Calavan and Oldfield 1979). The "yellows" diseases fall primarily into two symptom categories. In the first, caused by spiroplasmas and some phytoplasmas, symptoms result primarily from phloem dysfunction and possibly also from competition between the pathogen and the host plant for the phloem-borne photosynthates. They include wilting, stunting (shortened internodes), foliar chlorosis, die-back, occasional leaf yellowing or reddening, phloem necrosis and collapse of the sieve tubes and companion cells, callose deposition in the phloem elements, plant decline and death. A second type, caused by another group of phytoplasmas, involves plant growth regulator imbalances that result in often-distinctive deformations and overgrowths. The latter include early development and proliferation of axillary shoots (witches' brooming), bunchy top growth, and swollen veins (Figure 6). Flowers may be partially or completely sterile, and may exhibit phyllody (petals assuming a leaf-like form), asymmetry, virescence (petal greening) or "breaking" (irregular distribution of petal pigment) (Figure 7). In one case, the production of poinsettias with variegated bract pigmentation, the latter symptom actually is considered a positive quality. Symptom type and severity will vary with the pathogen, environmental conditions and age of the plant at the time of infection. Economic devastation may result from reduced product quality or partial to complete yield loss.
|
|
Figure 6. |
Figure 7. |
Critical to effective management of plant diseases are accurate diagnosis, pathogen detection and pathogen identification. Symptomatology can be an effective means of preliminary diagnosis for some mollicute diseases, particularly for those characterized by significant growth irregularities such as stunting, organ deformation and petal color changes. Phloem discoloration and/or necrosis (Figure 8) also may signal the presence of a vascular pathogen. More definitive means of identifying the causal agent, however, are necessary to confirm the etiology of the disease. Any of the three recognized phytopathogenic spiroplasma species (S. citri,
S. kunkelii and
S. phoenecium) can be cultured in specialized media (Figure 9), but phytoplasmas continue to resist cultivation. In the 1970s and 1980s convincing associations of phytoplasmas with many diseases were established by electron microscopic studies in which the pathogens were demonstrated in high numbers in sieve elements of symptomatic, but not healthy, plants. Light microscopy of sectioned plant tissues was employed in combination with the application of Dienes' stain, which colors the mollicute-colonized sieve tubes (Figure 10), and fluorescence microscopy was adapted for tissues stained with the nucleic acid stain, DAPI. More recently, as antisera to a number of phytopathogenic mollicutes became available, ELISA and immunoblotting techniques were successfully adapted. Currently, molecular tagging methods (DNA probes and primers) of varying sensitivity and specificity are used in most studies, and in the case of phytoplasmas, are often supplemented with RFLP pattern analysis to discern phylogenetic placement and to establish relationships of the disease agent with known mollicutes.
|
|
|
Figure 8 |
Figure 9. |
Figure 10. |
Mollicute-plant host interactions and the underlying physiology that results in the development of symptoms are not well understood. Although mollicutes evolved from walled bacterial ancestors, genes for virulence mechanisms found in such bacteria may or may not be present in the reduced genomes of mollicutes. Different factors may operate in different mollicute-plant systems, as evidenced by the two symptom categories mentioned above. Since the site of colonization in the plant host is the phloem sieve tubes, it is logical to suspect that phloem disruption could be a factor in symptom production and plant unthriftiness.
However, since the plant pathogenic mollicutes often cause disease in their leafhopper vectors and, in fact, may have originated as insect pathogens that invaded a new niche when introduced into plant phloem by feeding insects), it is possible that disease determinants operating in the insect may also be instrumental in producing plant distress.
Possible virulence-related factors include:
-
Phloem dysfunction. Spiroplasmas and phytoplasmas multiply to dense populations within many phloem sieve elements, undoubtedly utilizing a large fraction of the photosynthates being transported therein to the growing points of the host plant. Such competition takes a toll on the growth rate, height, and production of flowers and fruit. In addition, although the mollicutes can and often do migrate with the flow of phloem sap through the sieve tubes, is also is likely that they cause some physical blockage of the phloem, interrupting or inhibiting nutrient dissemination. Callose deposits within colonized sieve tubes also may contribute to the blockage of these conduits. The phloem sap of coconut palms suffering from the phytoplasma induced disease, lethal yellowing, was more concentrated but had a lower osmotic pressure than that of comparable, but healthy, trees.
-
Toxins. There is some evidence that spiroplasmas produce and export a translocatable phytotoxic substance that may contribute to the degeneration of colonized phloem cells. A low molecular weight (< 300) polar, acidic substance caused necrosis in a bean leaf assay, but its instability
in vitro has prevented in-depth characterization.
-
Hormones and growth regulators. Mollicute-induced leaf, stem and flower deformities, premature and/or indeterminate flowering, phyllody, virescence, and fasciation strongly suggest that growth regulators, produced either by the plant or by the mollicute, are out of their normal balance. A plant shoot tip culture experimental system determined that plant infection with either the aster yellows phytoplasma or
S. citri alters the normal levels of endogenous cytokinin and auxin. Premature flowering caused in periwinkle by the beet leafhopper-transmitted virescence agent could be mimicked by exogenous applications of kinetin.
-
Enzymes. Mollicute-induced plant diseases do not normally exhibit symptoms of soft rotting, and there is no experimental evidence for the production of cellulases or pectinases by phytoplasmas or spiroplasmas. Other enzymes, however, may mediate disease development. Differences in the levels of esterases (which digest suberins in potato scab) and RNases between pathogenic and nonpathogenic strains of
S. citri have been found.
-
Metabolic processes. Yellows-type symptoms could reflect physiological changes and effects on components or regulators of metabolic pathways. Isolated transposon mutants of
S. citri failed to cause symptoms in test plants and had a reduced multiplication rate
in planta. The transposon had integrated into a single site in the fructose operon, in a gene encoding a protein having homology with a repressor of the
E. coli deoxyribonucleotide operon, suggesting that fructose metabolism may in some way be linked to the spiroplasma disease process.
-
Plasmids. Almost all species of spiroplasmas that have been surveyed for the presence of plasmids contain them. Plasmids of pathogenic bacteria often contain virulence-related genes; however, the functions of these mollicute extrachromosomal DNA elements, which range in size from 2-39 kb (Razin et al.. 1998), are mostly unknown. One spiroplasma plasmid has been associated with resistance to erythromycin.
-
Viruses. Spiroplasma viruses, which range in morphology from rod-shaped to phage-like, are prevalent in spiroplasmas and may play both direct and indirect roles in the fitness of their hosts (Razin 1998). Directly, of course, the viruses may replicate and lyse the host cells, forming plaques on lawns of the bacteria. The state of the virus (lytic or lysogenic) may influence the ability of the spiroplasma to colonize, thrive in and cause disease in its host plants or vector insects, and some consideration has been given to the use of viruses in biological control of these pathogens. An indirect role for spiroplasma viruses in the host cell's fitness arises from the propensity of the viral nucleic acid to integrate randomly but frequently, as either an entire viral genome or as fragments, into the spiroplasma's genome. These insertions can inactivate genes or gene regulators, altering the biology of the spiroplasma, and they also provide a mechanism for the frequent and significant genome rearrangements and deletions that lead to the unexpectedly high rate of evolutionary change and niche adaptation in these wall-less prokaryotes.
-
Host responses. Periwinkle plants inoculated with
S. citri,
Candidatus Phytoplasma aurantifolia and the stolbur phytoplasma differentially expressed at least 24 cDNAs of genes related to photosynthesis, sugar translocation, stress responses, and synthesis of sterols. Correlations between particular cDNAs and certain disease symptoms were made, although the expressions were not unique to particular mollicutes.
Dissemination
Plant pathogenic mollicutes are transmitted by leafhoppers, planthoppers or psyllids (Class Insecta: Order Hemiptera: Suborder Homoptera). These small insects feed by inserting straw-like stylets into mesophyll, xylem, or phloem tissues of a suitable plant host. Gentle penetration of the phloem sieve element permits these insects to ingest phloem sap for up to several hours, during which they may acquire spiroplasmas or phytoplasmas from infected plants. Several factors may influence an insect species' ability to function as a mollicute vector, including propensity to feed from the host plant, ability to perform sustained phloem ingestion, and suitability as a spiroplasma or phytoplasma multiplication host.
Spiroplasmas and phytoplasmas have a propagative relationship with their vectors (Fletcher et al.. 1998). After being taken up by the feeding insect during phloem ingestion they move into the body cavity via the midgut region of the intestine. They are thought to move into insect cells via a receptor-mediated internalization event, as shown for
S. citri (Figure 11). Once inside gut epithelial cells the mollicutes multiply and then move across the plasmalemma and basal lamina into the hemocoel. Using hemolymph as a multiplication and transport medium, the pathogens migrate to the salivary glands, from which they are ejected with saliva into phloem during subsequent phloem probing. The entire transmission process can take 2-4 weeks, depending upon the titer of the source and duration of the acquisition feed. Plant pathogenic mollicutes are not known to be transmitted to the progeny (transovarial transmission).
|
Figure 11. |
S. kunkelii is transmitted in nature by a specific group of maize-colonizing leafhoppers in the genus
Dalbulus. The primary field vector of
S. kunkelii is
D. maidis (Figure 12), the corn leafhopper. Corn stunt is can be devastating to corn-growing regions in central Mexico and, because of the migratory range of
D. maidis, occasionally in the southern regions of the U.S. Other vectors include
D. elimatus, a leafhopper restricted to the higher elevations of central Mexico, and
Macrosteles quadrilineatus, an important experimental, but not a natural vector. Transmission efficiency can be quite high; 90-100% of acquiring
D. maidis can eventually transmit the pathogen.
S. citri occurs in all major citrus growing regions of the world. In the United States, the primary field vector is the beet leafhopper,
Circulifer tenellus, whereas in Europe Circulifer congeners and
Scaphitopius spp. are more important field vectors. Unlike the
S. kunkelii vector,
D. maidis, which is monophagous on maize,
C. tenellus has a broad host range and can feed on a number of different families of plants, which partially explains why
S. citri has a number of plant hosts from different plant families.
Phytoplasmas are transmitted in the same manner as spiroplasmas, but their vectors are not limited to the leafhoppers. Some phytoplasmas, including the causal agents of coconut lethal yellowing and pear decline, are transmitted by cixiid planthoppers and psyllids, respectively. Leafhoppers transmit phytoplasmas efficiently; for example,
D. maidis, the vector of maize bushy stunt phytoplasma, transmitted to 60-80% of the test plants. Other leafhoppers,
Macrosteles quadrilineatus and
Scaphoides titanus, are efficient vectors of the aster yellows and flavescence doree phytoplasmas, respectively. There is increasing evidence that phytoplasma infection may be beneficial to vector insects. Insects carrying the maize bushy stunt or aster yellows phytoplasma had higher survival rates than did mollicute-free control insects, especially at lower temperatures, supporting the hypothesis that phytoplasmas may have evolved in insects and moved secondarily to plant hosts.
Interestingly, aphids apparently do not serve as spiroplasma or phytoplasma vectors. Several species of aphid feed from the same host plants and perform sustained phloem ingestion, presumably ingesting spiroplasmas and phytoplasmas as would their leafhopper counterparts, but they have never been shown to acquire and/or transmit mollicutes. It is possible that aphids lack the ability to internalize phytoplasmas at the gut or salivary glands, or that phytoplasmas are incapable of multiplying in aphid hemocoel due to immune responses in the insect or to an inability of the mollicute to metabolize carbohydrates in the aphid hemolymph. However, neither of these hypotheses has been tested empirically.
Epidemiology and Management
Management strategies for spiroplasma and phytoplasma induced plant diseases can be divided into those directed at the pathogen and those directed at the vectors. Pathogen-based methods include resistant plant cultivars, but these are known for only a few host species. One example is the Maylayan dwarf palm, which is planted frequently in popular tourist areas where coconut lethal yellowing is prevalent. Genotypes of ash with tolerance to the ash yellows phytoplasma also have been identified. Chemical bactericides that inhibit cell wall formation, such as penicillin, are of no use for control of the wall-less mollicutes, but tetracyclines and others that influence the physiology of the prokaryote may limit pathogen titers in the plant (McCoy 1982). Tetracycline relatives have been used to preserve specimen trees and shrubs in landscape settings, but are not viable options for food plants because of expense and concern for pesticide residues in food products. Mollicutes have been eliminated from certain valuable plant materials by heat or CO2 therapies, or by meristem tip culture. Management options directed at the insect vectors are difficult because effective control can be accomplished only if applications precede insect migration into the fields. If chemicals are applied after vector species are detected in the crop, inoculative insects are likely to introduce the pathogen into host plants before a grower is aware of the insects' presence. Effective programs for aster yellows in muck-grown carrots and lettuce in the upper Midwest are based on nation-wide monitoring of the migration of
M. quadrilineatus, the primary vector of this phytoplasma in the United States.
Fastidious Phloem-Colonizing Bacteria
History
A subset of the plant diseases characterized by "yellows" type symptoms (foliar chlorosis, stunting, unthriftiness and death) and also suffering phloem necrosis, visible as a darkened ring in freshly cut stem sections, are incited by walled bacteria. These diseases have long presented challenges in diagnosis and management. Inability to consistently cultivate a pathogenic microbe from affected plants often led to mistaken attribution of causality, first to plant viruses and later, after plant pathogenic mollicutes were described, to phytoplasmas. Although most of these agents still resist cultivation in artificial medium our understanding of their nature and diversity has grown considerably, thanks to recent molecular characterization methods.
Biology
Phloem-resident walled bacteria are generally very small bacilli that generally possess Gram-negative prokaryotic cell morphology. The outer membranes of some are wavy or rippled in appearance, a feature that gave rise to the early name "Rickettsia-like organism" or RLO. As scientists worldwide gain access to physical and molecular tools such as electron microscopy, 16S rDNA sequence analysis and PCR, the number of diseases attributed to phloem-colonizing walled bacteria grows. In his recent review, Davis (2001) lists twenty four diseases in monocots and dicots, herbaceous plants and trees, vegetables, fruits and grains, and ornamental plants. They have been reported from the United States, Canada, the Caribbean, Central and South America, Europe, Asia, Africa, the Middle East and the Mediterranean countries.
Only a few of the phloem-resident bacterial plant pathogens have been placed taxonomically, and these fall into the phylum
Proteobacteria.
Candidatus Liberobacter asiaticum and
Candidatus L. africanum, causal agents of the very serious citrus greening disease in Asia and Africa, respectively, resemble the
Proteobacteria α-2 subgroup. The uncultivated agent of papaya bunchy top falls into the K-1 subgroup of the same family. The pathogen of a relatively recently recognized, but economically important disease of cucurbits called yellow vine (Figure 13), was recently identified as
Serratia marcescens (Sm), a member of the γ-3
Proteobacteria. Unlike the other phloem-colonizing bacteria,
Sm is readily cultured on common bacteriological media and cucurbit-infecting strains have been shown to differ significantly from strains isolated from other ecological niches.
S. marcescens, a cosmopolitan microbe in food products, stored wastes, bodies of water, and soil, is often an innocuous saprophyte, but may cause illness in immunocompromised vertebrates (including humans) and insect species. Strains of
Sm inhabit the rhizosphere (root zone) of plants, producing beneficial effects as plant growth promoting rhizobacteria (PGPR). Because of their anti-fungal properties certain Sm strains are also being evaluated as potential biocontrol agents in agricultural applications. Yet, certain strains invade plant tissues and cause economically devastating diseases in agronomically important crops.
|
Figure 13. |
Since most of the phloem-resident walled phytobacteria are uncultivable in artificial media, most of our knowledge of the phylogenetic placement of this group of pathogens comes from molecular analysis of cloned regions such as the 16S rDNA. Pathogens of other significant diseases, such as clover club leaf, have not yet been taxonomically characterized. However, other tools have been used to characterize these bacteria. The papaya bunchy top bacterium, a 0.25-0.35 by 0.8-1.6 µm bacillus, is surrounded by a Gram-negative type cell wall that apparently lacks a peptidoglycan layer in the periplasmic space. The two
Candidatus Liberobacter species, while similar to one another, cause different symptoms in host citrus species and are differentially affected by environmental temperature.
Host-Pathogen Interactions
Until the 1980s, the best-studied of the phloem-degenerating bacterial diseases was clover club leaf. The disease was named for the delayed opening of young leaflets of
Trifolium incarnatum (crimson clover), which remained curled in a club-like shape. The pathogen infects a wide experimental host range in at least seven plant families, and additional symptoms often include a slight floral virescence. Extracts from infected plants contained chains of cells ("thalli") having undulatory motility distinctive for this group. A more economically important disease, citrus greening, causes major losses in Asia and Africa (da Graca 1991). Prominent symptoms can include veinal chlorosis and occasional mottling of leaves, with secondary leaf size reduction and upright petioles. Fruits are asymmetrical, miniature, bitter in flavor and often prematurely abcised. The disease name, "greening," is derived from the color of the fruits that remain on the tree, which fail to ripen within the normal period of time (Figure 14). Cucurbit yellow vine, a new disease first recognized in the late 1980s, causes devastating losses in production of watermelon, squash, pumpkin and cantaloupe, but has never been reported to affect cucumber in the field. Plants turn yellow and wilt, often in a rapid, total-plant collapse. Phloem necrosis is evident as a honey-brown discoloration. Originally thought confined to the cross-timbers region of southern Oklahoma and northern Texas, yellow vine disease has recently been identified in several other midwestern states and as far east as Tennessee.
|
Figure 14 |
Dissemination
As is the case for the mollicutes, the taxa of insects that transmit phloem-limited bacteria are diverse. The citrus greening agents (L. asiatum and
L. africum) are transmitted by the aphid-like psyllids,
Trioza erytreae (Asian greening) and
Diaphorina citri (African greening), respectively. The bacterium can be acquired in 30 min., but transmission requires a 7-10 day latent period (De Graca 1991), suggesting that it multiplies in its psyllid vector.
The relationship of phloem-inhabiting bacteria with leafhopper vectors is less-well studied. Papaya bunchy top vectors include the leafhoppers
Empoasca stevensii, but the nature of the pathogen-vector relationship is unknown. The clover club leaf organism is transmitted by the leafhopper
Agalliopsis novella, but not by closely related leafhoppers
Agallia constricta and
Aceratagallia sanguinolenta. This bacterium multiplies within its leafhopper vector in a propagative relationship.
Recently, the causal agent of cucurbit yellow vine,
S. marcescens, was found to be transmitted by the squash bug,
Anasa tristis, a true bug (Figure 15). This relationship is unusual because true bugs are not often identified as vectors of phloem-associated pathogens. Squash bugs can acquire the bacterium after 24 hrs and can transmit from 1-21 days post acquisition. The bacterium is present in the hemolymph of some, but not all, transmitters, which is consistent with a non-circulative (resides in the foregut, not hemocoel) relationship between the bacterium and its vector.
|
Figure 15. |
Control
Use of antibiotics to reduce or eliminate phloem-resident pathogens has had limited success. In an investigation of management of citrus greening disease, tetracycline injections or sprays reduced foliar and fruit symptoms 10-97% (da Graca 1991), but other antibiotics, such as penicillin, were less effective. Thermotheraphy (heat treatment) can be effective, with up to 100% control when budwood from infected trees was heated to 47 C for 4 hr. However, this method is considered impractical for large scale use (da Graca 1991). No practical treatments are available for herbaceous plants infected with these bacteria.
Reducing pathogen dissemination by the use of vector-resistant varieties provides the best opportunity for long-term control of phloem-affecting diseases. However, in cases of acute vector infestations, insecticidal treatment is often the most effective management strategy. Use of natural enemies and the impact of insecticide treatment upon parasitoids and predators also must be considered in long-term management strategies.
In studies of cucurbit yellow vine, exclusion of the squash bug vector from susceptible plants and use of trap crops to prevent or reduce squash bug infestation resulted in reduced disease incidence. The most effective control of this disease, however, is the application of insecticides for control of squash bug populations. There is some progress in the development of
S. marcescens-resistant watermelon varieties.
History
Walled bacterial pathogens also inhabit the water-transporting cells of the xylem of their host plants and are transmitted by xylem feeding sharpshooters and spittlebugs, members of the leafhopper family (Raju and Wells 1986). Once assumed to be viruses, these bacteria were later designated "fastidious" because of the difficulty of cultivation, and "rickettsia-like" because of superficial similarities of their rippled walls with that group. The XLB now include a number of cultivated members that cause various diseases in a wide range of plant hosts.
Clavibacter xyli subsp.
xyli (sugarcane ratoon stunting disease) and C.
xyli subsp.
cynodontis (bermudagrass stunting disease), two examples of xylem-limited bacteria, are fastidious, slow-growing Gram-positive bacilli of variable form that often appear coryneform (club-shaped) and often occur in pairs linked at one end to form a V-shape (Davis and Vidaver 2001). Other Gram-positive forms include several pathovars of
Curtobacterium flaccumfaciens that cause vascular diseases of beets, beans and soybeans, tulips and poinsettias (Davis and Vidaver 2001).
Xylella fastidiosa, the best studied of the XLB, has a wide host range and causes economically significant damage in most of its plant hosts. Pierce's disease of grapevines has occurred in California for years, and has recently been identified in Europe. Phony peach, leaf scorches of oleander, almond and several forest trees, and a leaf scald of plum are other examples of diseases caused by this bacterium in California, Georgia and other U.S. states. In Taiwan, pear leaf scorch has been attributed to
X. fastidiosa, and in Brazil and Argentina, X. fastidiosa-caused citrus variegated chlorosis (Figure 16) has become a major limitation to citrus production, and the same pathogen was identified as the causal agent of a leaf scald of coffee. In each of these diseases, the bacterium is transmitted from plant to plant by insect vectors, particularly members of the sharpshooter family. Pierce's disease of grapes was long considered to be a relatively minor disease in California, as its vector, the blue-green sharpshooter, was relatively sedentary. However, this disease has recently taken on a much more devastating role, threatening the grape and wine industries in that state. The change in importance of PD comes not from a mutation in bacterial virulence genes, or from the widespread planting of a susceptible grape cultivar, but rather to the invasion of the area by an invasive insect, the glassy-winged sharpshooter,
Homolodisca coagulata (Figure 17). Acquiring
X. fastidiosa as it feeds on the ubiquitous grapevines in southern and central California, this insect moves rapidly and over long distances, feeding as it goes and efficiently disseminating the bacterium plant to plant and vineyard to vineyard.
|
|
Figure 16. |
Figure 17. |
Biology
X. fastidiosa is a small, narrow, Gram-negative bacillus that grows slowly on specialized media (Hopkins 2001). Strains and pathotypes of the species have been identified and characterized through a number of molecular methods including rep-PCR, RAPD-PCR, RFLPs and DNA-DNA hybridization. The host-pathogen interactions leading to disease symptoms are not well understood, but most likely it is the occlusion of xylem vessels by high bacterial populations that leads to plant death.
X. fastidiosa was the first plant pathogenic bacterium for which the complete genome was sequenced, work done by a team of Brazilian scientists working together as a consortium. Others have since derived valuable information from the sequence itself as well as from alignments with those of other microorganisms (Dow and Daniels 2000). These remarkable accomplishments have not yet resulted in the development of a genetic system in which to study pathogenicity and virulence characteristics, but native plasmids isolated and characterized from several strains of the bacterium may be useful as vectors for bacterial transformation.
Host-Pathogen Interactions
Host-Pathogen Interactions
X. fastidiosa was originally thought to infect only a few woody plants, including grapevines. As detection technology advanced, more diseases of unknown etiology were attributed to
X. fastidiosa, and the host range now includes over 75 plants, some of which are listed in (Table 1).
Table 1. Some plant hosts of, and diseases caused by,
X. fastidiosa.
PLANT |
DISEASE |
Grapevine |
Pierce’s disease |
Citrus |
Citrus variegated chlorosis |
Oleander |
Oleander leaf scorch |
Almond |
Almond leaf scorch |
Oak |
Oak leaf scorch |
Sycamore |
Sycamore leaf scorch |
Alfalfa |
Alfalfa dwarf |
Peach |
Phony peach |
Symptoms of Pierce's disease of grapevine can be detected 3-15 months after initial infection and usually include 1) scalding, progressive drying of the leaf from the margin toward the leaf petiole, 2) browning of the scalded areas, 3) dwarfing, wilting, or withering of part or all of the vine, 4) gradual death of the root system, 5) death of the vine within 3 to 48 months (Figure 18). In sweet orange trees, citrus variegated chlorosis symptoms include conspicuous areas of variegation on the upper side of older leaves and raised lesions on the underside of the leaves. Fruit are small and hard. Leaf scorch of landscape and fruit trees is typified by an irregular marginal or interveinal necrosis that expands throughout the leaf causing deformation and early defoliation. Many plant hosts are symptomless.
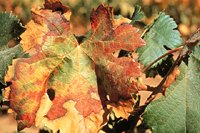 |
Figure 18. |
The symptoms associated with
X. fastidiosa infection suggest that the water-conducting function of xylem is disrupted by the presence of the bacteria. Bacterial aggregates, gum deposits or tyloses that block tracheary elements all contribute to water stress in the infected plant. Interestingly, electron micrographs of affected plants show variation in the number and types of occluded xylem elements, leading to some disagreement over the mechanisms involved in disease development. One theory is that a phytotoxin released by the pathogen is responsible for leaf scorching. Another possibility is that
X. fastidiosa infection affects the balance of growth regulators. Applications of gibberellic acid to peach trees infected with phony peach disease show some remission of symptoms.
Dissemination
X. fastidiosa is transmitted by xylem-feeding homopterans. This group of insects includes the sharpshooter leafhoppers (subfamily Cicadellinae), and the spittlebugs (Family Cercopidae). It is possible that cicadas, which also feed from xylem, can transmit
X. fastidiosa, but this has not yet been demonstrated.
Several species of sharpshooters are natural or experimental vectors of
X. fastidiosa in its interactions with grapevines, but only three are considered to be of economic importance: the green sharpshooter,
Draeculacephala minerva, the blue-green sharpshooter,
Graphocephala atropuntata, and the glassy-wing sharpshooter,
Homalodisca coagulata. Of these three species, the glassy wing sharpshooter (GWSS) is considered to be the most threatening because of its recent introduction and rapid proliferation in grape and citrus growing areas in California.
Although the GWSS was previously only a minor vector of
X. fastidiosa, the host range of this insect has recently been shown to include grapes, oleander, citrus, and a number of other woody plants. The species is capable of overwintering under certain conditions and may harbor
X. fastidiosa for extended periods of time. It is also capable of feeding on dormant woody tissues, from which it can acquire the pathogen.
The relationship between
X. fastidiosa and the sharpshooter leafhoppers is unusual. Bacteria taken up by the feeding insect colonize the food canal within the straw-like stylets, which are used to pierce plant tissues. Bacteria adhering to the insect cuticle grow into biofilms that line the inner surface of the food canal. Adults and nymphs are capable of transmitting
X. fastidiosa immediately after acquisition, but nymphs lose the ability to transmit after molting. An adult acquiring X. fastidiosa can retain the ability to transmit for several months to life of the insect.
Epidemiology and Management
X. fastidiosa is considered one of the world's emerging plant pathogens because of its potential economic impact on two major agricultural commodities, citrus and grapes. To a lesser degree it also affects the ornamental industry. Management of this pathogen is approached on two fronts: control of the vector and development of resistant plant varieties.
Acute infestations of sharpshooters may be controlled by chemical treatment of host plants; both topical and systemic insecticides have proven somewhat effective in reducing sharpshooter populations. However, public opposition to pesticide use for eradication has been considerable in the past. Biological alternatives to synthetic pesticides include parasitic wasps and entomopathogens, but historically these have not been effective against leafhoppers as a means to control plant disease. Management of
X. fastidiosa and its vector(s) is a topic of extremely active current research, especially in the vineyard regions of California where Pierce's disease threatens the economic health of the industry.
Long term control strategies must include development of disease resistant plants, a challenging prospect since many
X. fastidiosa-susceptible plants are perennial. A novel approach to control of this devastating disease may be through genetic manipulation of the non-Xylella enteric bacteria that live naturally in the gut of leafhoppers. Using the process of
paratransgenesis, researchers hope to transform normal gut bacteria to secrete a toxin lethal to
X. fastidiosa. The transformed bacteria would be passed from insect to insect via normal copraphagous activity (ingestion of fecal matter), negatively affecting
X. fastidiosa as it is acquired by the paratransgenic insects.
Conclusions
The plant vascular-inhabiting bacteria, walled or wall-less, are a diverse group of phytopathogens that often maintain complex and intimate relationships with their insect vectors as well as with their plant hosts. Despite their fastidious natures or, in some cases, obligate parasitism, new research strategies that do not require microbial cultivation have allowed significant progress in unravelling the behavioral and molecular mechanisms by which these prokaryotes invade, colonize and impact the growth and productivity of their hosts. These promising areas of research will certainly facilitate the development of more effective detection and identification tools as well as highlighting weak points in the disease cycles that will allow significant improvements in our ability to moderate the effects of these organisms on our food, fiber and ornamental crops.
Acknowledgments
We thank S. Von Broembsen and L. Littlefield for critical review of the manuscript. This is a publication of the Oklahoma Agricultural Experiment Station, Project # OKLO 2052.
References
Calavan, E.C. and G.N. Oldfield. 1979. Symptomatology of spiroplasmal plant diseases. Pp. 37-64 in Whitcomb, R.F. and J.G. Tully, eds. The Mycoplasmas, Vol. III. Academic Press, Inc., New York.
da Graça, J.V. 1991. Citrus greening disease. Annu. Rev. Phytopathol. 29:109-136
Davis, M.J. 2001. Fastidious phloem-limited bacteria. Pp. 275-282 in Laboratory Manual for the Identification of Plant Pathogenic Bacteria; 3rd Edition. N.W. Schaad, J.B. Jones and W. Chun, eds., APS Press, Inc., St. Paul, MN
Davis, M.J. and A.K. Vidaver. 2001. Coryneform plant pathogens. pp. 218-235 In: N.W. Schaad, J.B. Jones and W. Chun.
Laboratory Guide for Identification of Plant Pathogenic Bacteria. 3rd Ed. APS Press, Inc., St. Paul, MN.
Dow, J.M. and M.J. Daniels.2000.
Xylella genomics and bacterial pathogenicity. Yeast 17:263-271.
Fletcher, J., A. Wayadande, U. Melcher and F. Ye. 1998. The phytopathogenic mollicute-vector interface: a closer look. Pytopathology 88: 1351-1358.
Hackett K.J. and T.B. Clark. 1989. Spiroplasma ecology. Pp. 113-200 in Whitcomb, R.F. and J.G. Tully, eds., The Mycoplasmas, Vol. 5. Academic Press, Inc.; New York.
Hopkins, D.L. 1989.
Xylella fastidiosa: Xylem-limited bacterial pathogens of plants. Ann. Rev. Phytopathol. 27:271-290.
Hopkins, D. 2001.
Xylella fastidiosa. pp. 201-213 In: N.W. Schaad, J.B. Jones and W. Chun, eds.
Laboratory Guide for Identification of Plant Pathogenic Bacteria. 3rd Ed. APS Press, Inc., St. Paul, MN.
Lee, I.-M., R.E. Davis and J. Fletcher. 2000. The Mollicutes: Spiroplasmas and Phytoplasmas. In: N.W. Schaad, ed. Laboratory Guide for Identification of Plant Pathogenic Bacteria. 3rd Ed. APS Press, Inc., St. Paul, MN.
McCoy, R.E. 1982. Use of tetracycline antibiotics to control yellows diseases. Plant Dis. 66:539-542.
Raju, B.C. and J.M. Wells. 1986. Diseases caused by fastidious xylem-limited bacteria and strategies for management. Plant Dis. 70:182-186.
Razin, S., D. Yogev, and Y. Naot. 1998. Molecular biology and pathogenicity of mycoplasmas. Microbiol. and Molec. Biol. Rev. 62:1094-1156.
Sears, B.B. and B.C. Kirkpatrick. 1994. Unveiling the evolutionary relationships of plant-pathogenic mycoplasmalike organisms. Am. Soc. Microbiol. News 60:307-312.